CHAPTER 10
ROCKETS AND SPACECRAFT: SINE QUA NON OF SPACE SCIENCE
Even as NASA was being formed, the stable of American sounding rockets was impressive. There were small (Deacon, Cajun, Arcon, Arcas), medium (Aerobee, Aerobee-Hi), and large (Viking) rockets. Viking had been designed to replace the V-2, which was no longer used after the test program ended in 1952. There were rockets using solid propellants (Deacon, Cajun, Arcon, Arcas) and rockets using liquid propellants (second stage of Aerobee, Viking). Multistage combinations (Nike-Deacon, Nike-Cajun, Aerobee) achieved higher altitudes than could economically be attained with single-stage rockets. Rockets had been launched from balloons, from aircraft, and from launchers aboard ships at sea. These sounding rockets and the high-altitude research program that went with them provided NASA with an immediately on-going component of its space science program.1
A similar situation existed with respect to the larger vehicles needed for launching spacecraft into orbit. The reentry test vehicle Jupiter C-which launched America’s first satellite, Explorer 1, and which used the Redstone missile as its first stage-gave rise to a first group of what were called Juno space launch vehicles. Later versions of Juno used the more powerful Jupiter intermediate-range ballistic missile as the first stage.2 The Redstone, which was created for the Army by the von Braun team and in which one could detect a distinct V-2 ancestry, was on hand and was used for America’s first suborbital manned flights.3 The Vanguard IGY launch vehicle, which used derivatives of the Viking and Aerobee sounding rockets as its first and second stages, was also available.4 NASA took over Vanguard from the Naval Research Laboratory and completed the program, after which the Vanguard first stage was retired; but the upper stages were combined with the Air Force’s Thor to create the Thor-Delta, or simply Delta, launch vehicle, which from the very start was one of the most useful of the medium-sized combinations.5 In 1958 the Air Force’s Atlas was the most powerful U.S. rocket that could be quickly pressed into service as a space launcher. To it was assigned the launching of The first American astronauts to go into orbit.6 Atlas eventually became the main stage of Atlas-Agena and Atlas-Centaur, multistage launch vehicles used to put multiton payloads into space.
The imposing presence of the Soviet Union in space following the launching of the first Sputniks and the substantial lead it apparently had over the U.S. in payload capability generated a sense of urgency to develop very large payload capabilities. But with the variety of vehicles already in its stable or imminent, the United States clearly was not going to have to start from scratch. Indeed, had the country been willing to use the von Braun rockets for the IGY satellite program, the first satellite in orbit could well have borne a “made in America" stamp. At any rate, even this partial survey of the situation at the time NASA got going shows how deep in the rocket and missile work of the 1950s lay some of the roots of the subsequent space program.
Of course, along with the missiles and rockets available to NASA and the military were associated facilities and equipment already in operation. Launch ranges existed in Florida, California, New Mexico, and Canada. Tracking and telemetering stations, strategically located in the U.S. and elsewhere, were working. Vanguard Minitrack network of radio-tracking and telemetering stations for operating with the IGY satellites spanned the globe and provided a nucleus on which to build for an enlarged program of the future. IGY optical tracking stations also girdled the globe and were immediately available for photographic and visual tracking of spacecraft that were large enough to be detected by such means. To produce all these a substantial component of American aerospace, electronics, and other industry had been employed, generating hardware and acquiring an experience ready to be used for tackling the challenges that lay ahead.7
One of the first tasks facing NASA in the fall of 1958 was to determine what additional launch vehicles would be required to accomplish the space missions planned for the program. While most of the launchers would derive from military hardware, some, especially for the manned spaceflight program, would have to be built from scratch. So, too, would the space craft for the science, applications, and manned spaceflight missions.
LAUNCH VEHICLES
It is not necessary for understanding the relationship of launch vehicles to the space science program to delve deeply into how they were developed, but a few principles should be understood. First, a number of different vehicles were required. One might have supposed that a single launch vehicle, which could do everything the program required, would be ideal. With only one manufacturing line, one kind of assembly, test, and launch facilities, one kind of operational equipment and procedures, and basically one launch team, a substantial background of experience would quickly build up for that vehicle. Engineers and technicians would become thoroughly familiar with its characteristics and idiosyncrasies, so that a high degree of reliability could be ensured.
But in an era when a launch vehicle was expended for each firing, the economics would not be favorable. For, to be acceptable, a single vehicle would have to be able to accomplish both the simplest and the most difficult of the missions required-from small, near-earth satellite missions to manned flights to the moon. On the most difficult missions, the launch vehicle would presumably operate most efficiently, and the costs would be commensurate with the accomplishments. But to use such a vehicle for less demanding missions would be most inefficient; indeed, the cost of the launch vehicle could overwhelm the cost of the spacecraft. To mitigate this problem of cost there would, of course, be pressure to fly many small missions on a single launch vehicle, or to let small missions ride piggyback on larger ones, thus, reducing the cost per spacecraft; but then different kinds of complications would enter in. Some of these would be fundamental, as when one set of experiments required a circular orbit, another set an eccentric orbit, still another a polar orbit, and a fourth an equatorial orbit.
For expendable launch vehicles such considerations led to the conclusion that the most efficient approach would be a graduated series running from a small, inexpensive vehicle to the very large, very expensive ones. The gradation between vehicles would be large enough to yield a substantial increase in payload and mission capability, but small enough to avoid having to use vehicles too costly for their assigned missions. Obviously the way in which these requirements were met was a matter of judgment, and in some respects arbitrary. The subject was constantly under study by both the military and NASA.8
Second, the basic physics of rockets dictated that major launch vehicles should be multistage, or step, rockets-that is, combinations of two or more rockets, called stages, which burn one after the other. As soon as the first stage has used its propellants, it is discarded, after which at an appropriate time the second stage is ignited. When the second stage has burned out and been discarded, the third stage is ready to fire. And so on. Multistaging is important for rockets that must work against the force of gravity, for otherwise the propellants must supply the energy needed to propel the entire rocket structure against the pull of gravity for the whole launching phase. But with staging, in which portions of the structure are discarded as soon as they are no longer needed, only a small fraction of the entire vehicle need be propelled into the final orbit or space trajectory. The early rocket pioneers recognized the importance of staging, a point that Robert Goddard elaborated in his famous Smithsonian paper.9 In the space program two-stage and three-stage vehicles became common, four-and five-stage combinations not uncommon.
In the scramble to put together a national launch vehicle capability after the formation of NASA, it was natural that whatever vehicles were available or could be assembled from the existing military programs would be used. In 1959 six out of the seven vehicles that were available to NASA came largely from the missile program.10 The seventh was Vanguard, which had been built by the Navy for the IGY.
To expand the national capability, additional vehicles would be developed (fig. 10). Scout, a four-stage, solid-propellant vehicle, would provide an inexpensive means for launching 70 kilograms to 185 (or even 550) kilometers. Vega and Centaur, the latter using the high-energy propellants liquid hydrogen and liquid oxygen, would substantially extend the launch capability of Atlas-based vehicles. Juno V vehicles and Nova were intended to support a variety of manned missions. Nova was expected to generate a thrust of 6000000 pounds-almost 27,000,000 newtons-and would be required for launching men in a direct ascent from the ground to the moon’s- surface.
Fifteen years later the situation was entirely different. By then a bewildering variety of rocket stages and launch vehicle combinations had been developed, along with an extensive literature.11 What the vehicles could do for the various Space missions can be deduced from the performance figures for the launch vehicles in figures 10 through 14.
As seen in figure 11, by 1962 the Hustler and Vega had been eliminated. Likewise, Juno II, which had not proved particularly useful, had beer dropped. Nova plans called for doubled thrust, and Saturn was to rely on liquid-hydrogen, liquid-oxygen engines for its upper stages. By 1966 Now had disappeared because of NASA’s decision in July 1962 to use lunar-orbit rendezvous instead of direct ascent for the Apollo missions.12 Several versions of Saturn would support NASA’s manned spaceflight programs; the largest, Saturn V, would be used for the manned lunar missions.13 The Department of Defense preferred not to be tied into the expensive and highly experimental Saturn, so in following years the Titan III line of launchers was introduced into the stable to support large-scale military missions. Titan III additions can be seen in the display for 1972 (fig. 13), at which time the only Thor-based vehicle remaining was Delta.
These launch vehicles, with the sounding rockets discussed at the start of this chapter, made up the backbone of U.S. capability to explore and investigate space. They resulted from joint planning by NASA and the military to serve their respective needs.14 Other rockets and rocket stages were put together for special purposes, usually by the military, but their existence did not change the overall picture.
In the 1970s a basic change was initiated with the commitment to the Space Shuttle, which in the 1980s would supplant most of the expendable boosters for launching spacecraft into near-earth orbit. The launch vehicle line-up in the fall of 1976 (fig. 14) shows how the elimination of the Saturns, following the completion of the Apollo and Skylab programs by the mid-1970s, and the prospect of the Space Shuttle by the 1980s had thinned out the stable. Only five vehicles remained: Scout for small payloads, Delta and Atlas-Centaur for medium and large payloads, and two Titan III combinations for the very large payloads.
Throughout the entire evolution of the launch vehicle stable, both Scout and Delta remained. Relatively inexpensive, able to support a substantial number of the researches that scientists wanted to do, these launch vehicles had great appeal. Even with the Shuttle in operation, Scout at least was likely to remain, for even the Shuttle might not prove economical for small missions with a wide variety of special trajectory requirements.
Scout and Delta also illustrate another feature of the national launch vehicle program. As the group of vehicles improved as a family over the years, performance of the individual vehicles also improved. In 1962 Scout could put 100 kg into a near-earth orbit; by the 1970s this performance had doubled. In the same period Delta’s performance had shown an even greater growth. In 1962 Delta could send several hundred kilograms into a near-earth orbit or 25 kg to Mars or Venus; by 1976 Delta could loft 2000 kg into a 185-km orbit or 340 kg to the near planets. The increased performance, which most vehicles experienced over the years, was brought by continuing programs of improving and uprating the vehicles. While improvement programs were the pride of the vehicle engineers, they were sometimes the bane of top management, which would often have preferred to settle upon some acceptable level of performance and then stop spending any more money on vehicle development.
Like the United States, the Soviet Union developed a launch vehicle stable.15 During the 1960s, however, the Soviet Union appeared to rely on fewer kinds of vehicles than did the United States, preferring to use a single model for a wider variety of missions. The Russian preference may be prima facie evidence that the economic factors of using large vehicles for small-payload missions were not as prohibitive as American planners felt they were; but comparing Russian economics with American is a risky business. The USSR may simply have decided to pay the extra cost for the convenience afforded.
Only the United States and the Soviet Union developed extensive launch capabilities. Other nations interested in space research and applications turned largely to the United States for assistance in launching spacecraft or individual experiments, as will be explored in chapter 18. Some nations, however, desiring to lessen this dependence, proceeded to develop vehicles of their own. Among those were Britain, France, Japan, People’s Republic of China, and the European Launcher Development Organization, a coalition of countries that pooled resources to develop a launcher approaching the Atlas-Agena capability.16 Italy set up an equatorial launching facility in the Indian Ocean off the coast of Kenya, but used the American Scout as launch vehicle.17
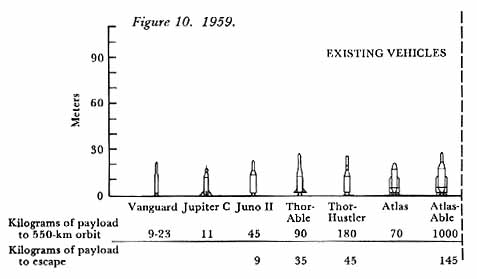
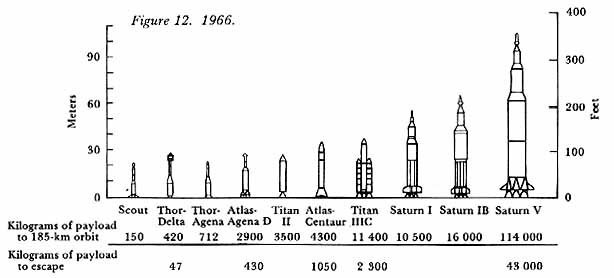
1959.
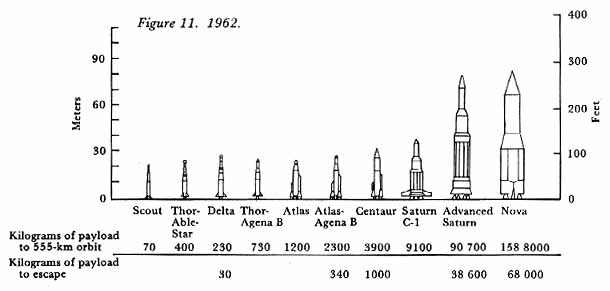
1962.
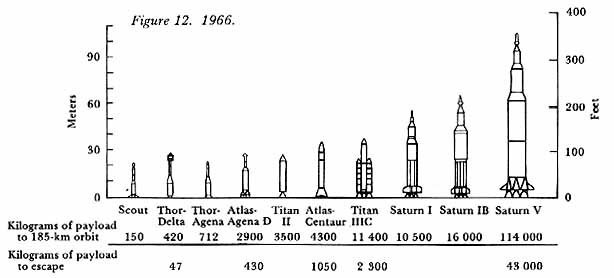
1966.
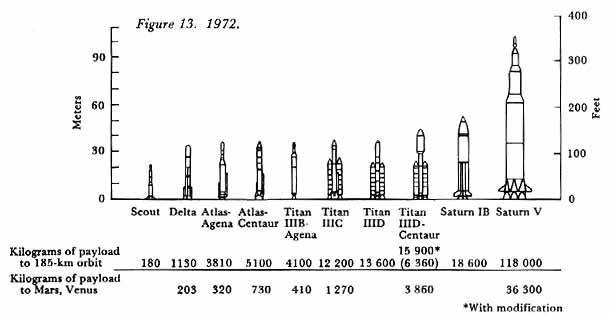
1972.
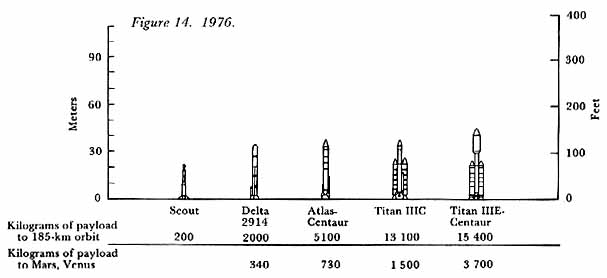
1976.
By 1966-when Centaur became fully operational-the United States could at last launch spacecraft for just about any space mission the country might want to undertake, except the very demanding ones requiring the Saturn or Titan still under development. Although the debate over whether the United States could or could not match Russian launch capability still arose occasionally, the subject no longer had the importance it once did. As long as the United States could carry out the scientific investigations and make the space applications it desired-sometimes using miniaturization techniques to overcome limitations on total payload weights-the country could compete with the Soviet Union on essentially equal terms, and the comparative sizes of rockets were then an artificial criterion on which to judge Soviet and U.S. space prowess.
The ability to launch objects and men into space rested on a substantial investment in manpower and facilities-design, engineering, construction, assembly, and test facilities in both industry and government. Most visible were the launching ranges, which along with the launch vehicles themselves symbolized. the nation’s space capability. In the United States the principal facilities were the Eastern Test Range, with launch areas at Cape Canaveral and Merritt Island in Florida; and the Western Test Range, for which the launching areas were at Vandenberg Air Force Base in California.18 A smaller launch station was used by NASA for firing Scouts from Wallops Island on the Virginia coast.19 Supplementing these were sounding rocket ranges at the White Sands Missile Test Facility in New Mexico, Wallops Island, Point Mugu in California, and Fort Churchill in northern Canada. Occasionally shipborne launchers were used for special missions.
The Soviet Union operated a number of major launch ranges out of Tyuratam, Kapustin Yar, and Plesetzk.20 A few other countries established satellite launch ranges.21 By invitation American sounding rockets were fired at numerous ranges around the world-for example, at Thumba in India, at Woomera in Australia, and at Andoeya, Norway.22
SPACECRAFT
What the United States could do with its launch capabilities was revealed by the spacecraft the country built and launched. As with the many rockets and rocket stages, one could easily become totally immersed in the subject of spacecraft, which displayed a bewildering variety of shapes, sizes, and purposes. A partial listing of U.S. and foreign spacecraft is given in tables 3 and 4.23 As with launch vehicles, it is not necessary to know all the spacecraft in intimate detail to understand their role in the space science program. A few general concepts suffice. By dividing spacecraft into a few representative classes, one can understand their various functions better.
United States Spacecraft
(Partial list, excluding commercial and Defense Department spacecraft)
Vanguard | International Geophysical Year, Explorer class. |
---|---|
Explorer | Small satellite for near-earth missions. |
Interplanetary Monitoring Platform (IMP) | Explorer-class satellite to explore cislunar and lunar space. |
Orbiting Geophysical Observatory (OGO) | Observatory-class satellite for geophysical research. |
Orbiting Astronomical Observatory (OAO) | Observatory-class satellite for solar studies. |
High Energy Astronomical Observatory (HEAO) | Observatory-class satellite for stellar astronomy. |
High Energy Astronomical Observatory (HEAO) | Very heavy, observatory-class satellite for studying shortwave and high-energy phenomena in cosmos. |
Biosatellite | Observatory-class, recoverable satellite for life sciences. |
Pegasus | Observatory-class satellite for micrometeoroid studies. |
Echo | Applications satellite. Large metallized sphere for passive communications studies. |
Relay | Active communications satellite. |
Syncom | Active communications satellite in synchronous orbit. |
Applications Technology Satellite (ATS) | Platform for a variety of applications technology researches, particularly in synchronous orbit. |
Tiros | Large Explorer-class weather satellite. |
ESSA | Operational version of Tiros. |
Nimbus | Observatory-class weather satellite. |
ERTS | Earth Resources Technology Satellite. Applications satellite devoted to earth resources research. |
Synchronous-orbit Meteorological Satellite (SMS) | Applications satellite in synchronous orbit for meteorological research. |
PAGEOS | Passive geodetic satellite. |
GEOS | Active geodetic satellite with flashing lights and radio instrumentation. |
LAGEOS | Geodetic satellite with comer reflectors for use with laser beams from the ground. |
Mercury | One-man satellite. |
Gemini | Two-man satellite. |
Apollo (earth-orbiting) | Three-man satellite. |
Ranger | Lunar hard lander. |
---|---|
Surveyor | Lunar soft lander. |
Lunar Orbiter | Lunar satellite for photography of the moon and lunar environment studies. |
Apollo lunar module with command module | Manned lunar lander with manned lunar orbiter. |
Pioneer | Explorer-class interplanetary probe. |
Mariner | Observatory-class planetary and interplanetary probe. |
Viking | Observatory-class planetary orbiter plus lander. |
Voyager | Observatory-class planetary and interplanetary probe for outer planet studies. (Spacecraft of the 1970s, not the Voyager of the 1960s that was displaced from the program by Viking.) |
Foreign Spacecraft
Sputnik | Geophysical research satellite. |
---|---|
Luna | Unmanned lunar orbiter, lander, and return missions. |
Vostok | First Soviet manned spacecraft. |
Voskhod | Adaptation of Vostok to accommodate two and three cosmonauts. |
Soyuz | Two- or three-man spacecraft, with working compartment. |
Salyut | Earth-orbiting space station for prolonged occupancy and revistation by cosmonauts |
Cosmos | Catchall name for variety of research and test spacecraft. |
Venus (Venera) | Unmanned Venus probe. |
Polyot | Earth satellite with onboard propulsion for changing orbits. |
Elektron | Radiation belt satellite, launched in pairs. |
Zond | Lunar and deep-space probe. |
Molniya | Communications satellite in 12-hour orbit with low perigee and with apogee near synchronous-orbit altitude. |
Meteor | Weather satellite. |
Intercosmos | Soviet international satellite. |
Oreol | Scientific satellite for upper atmosphere and auroral studies. |
Mars | Unmanned Martian probe. |
Prognoz | Satellite to study solar plasma fluxes. |
Raduga | Geosynchronous communication satellite. |
Ekran | Television broadcasting satellite. |
Ariel | United Kingdom satellite for geophysical and astronomical research. |
---|---|
Alouette | Canadian satellite for ionospheric research. |
Isis | Canadian satellite for ionospheric research. |
San Marco | Italian satellite for geophysical research. |
French (FR-1) | French satellite for ionospheric research. |
ESRO | European Space Research. |
ASUR | German satellite for particles and fields research. |
Skynet | United Kingdom satellite for communications. |
NATO | NATO satellite for communications. |
CAS (Eole) | French satellite for data collection and meteorology. |
Barium Ion Cloud | German satellite for geophysical research. |
ANIK | Canadian geosynchronous satellite for communications. |
Aeros | German geophysical satellite. |
ANS | Netherlands satellite for ultraviolet and x-ray astronomy. |
INTASAT | Spanish satellite for ionospheric research. |
Helios | German deep-space probe for interplanetary and solar studies inside the orbit of Mercury. |
Symphonie | French-German satellite for communications. |
COS | European Space Agency satellite for study of cosmic gamma rays. |
First, there was the sounding rocket, a rocket instrumented for high-altitude research and fired along a vertical or nearly vertical trajectory. To distinguish between sounding rockets and space probes, a limit of one earth’s radius was arbitrarily set on the altitude of a sounding rocket.24 The sounding rocket was really both launch vehicle and payload combined. Only rarely was the payload separated from the flying rocket, and when this did happen, the payload still traversed an up-and-down trajectory alongside that of its launching rocket.
Sounding rockets, which were the only high-altitude research vehicles capable of exceeding balloon ceilings before the launching of Sputnik, continued through the 1970s to be important in the U.S. space program, being launched at the rate of about 100 a year. They provided the best means of obtaining vertical cross sections of atmospheric properties up to satellite altitudes and also were inexpensive devices for trying out new instrumentation or making exploratory measurements of phenomena to be studied in detail later with more expensive spacecraft. Their relatively low cost and the speed with which a sounding rocket experiment could be prepared and carried out also made sounding rockets useful for graduate research where the student needed to complete a project in a reasonable amount of time to support his dissertation. But not only students found the sounding rocket attractive. Many professional space scientists continued to favor sounding rockets for much of their research, as opposed to the more complicated, more expensive, and more demanding satellites.25 Through the years there was a steady demand on NASA for sounding rockets, and the agency was frequently urged to increase its budget in this area, even though by 1965 the budget had risen to $19 million a year, an order of magnitude more than had been spent per year on such research during the days of the Upper Atmosphere Rocket Research Panel.
Yet satellites were required for many space experiments, particularly for long-duration observations above the earth’s atmosphere. For these, many different satellites were devised, as may be seen from table 3. Generally these spacecraft could be divided into three classes: Explorers, observatories, and manned spacecraft.
The simplest were the Explorers, whose weights usually ranged from less than 50 kilograms to several hundred (fig. 15). Each was devoted either to a single experiment or to a small collection of related experiments. Explorers were either unstabilized or used fairly simple techniques for obtaining a rough degree of stability. If the spacecraft were spun around a suitable axis, that axis would maintain its general direction in space. Long booms could be used to generate a gravitational torque on the satellite, keeping a chosen side toward the ground. Explorers usually used battery power-supplies, sometimes replenished by energy from solar cells.
Explorers were much simpler than observatory satellites. The observatory class-which included the Orbiting Solar Observatory (fig. 16), the Orbiting Geophysical Observatory (fig. 17), and the Orbiting Astronomical Observatory (fig. 18)- consisted of very heavy, complex, accurately stabilized spacecraft. Weights ranged from several hundreds of kilograms to tons. The much greater size and weight of the observatories permitted more scientific payload and more sophisticated instrumentation. They could devote a considerable weight to what was called “housekeeping" equipment-power supplies, temperature control, and tracking and telemetering equipment. As with the Orbiting Geophysical Observatory, there might be provision for special operations such as erecting booms to hold instruments like magnetometers at a distance from the main body of the spacecraft, which otherwise might influence the measurements to be made. The Orbiting Astronomical Observatory and the Nimbus meteorological satellite were equipped with large paddles, covered with solar cells, which could be unfolded and kept facing the sun to furnish power for the spacecraft and its instruments. As a rule observatories carried elaborate systems for maintaining a desired orientation in space, so that scientific instruments could be pointed in a chosen direction. This was especially true of the astronomical satellites, whose instruments measured radiations from selected celestial bodies. The Orbiting Astronomical Observatory, for example, used specially designed star trackers which, by fixing on several chosen stars in widely different directions, could establish a reference frame for the spacecraft. With this reference frame as a guide, the observatory could be slewed around to any chosen direction. Once properly oriented, the spacecraft could be held fixed to within a minute of arc, and telescopes within the satellite could be trained for long periods of time on their observational targets with an accuracy of fractions of a second of arc.
A variety of schemes provided the ability to alter and then maintain spacecraft orientation. Most frequently used were small intermittent jets, developed either by small chemical rockets or by releasing high-pressure gas-nitrogen, for example-through small nozzles. Other methods were also used, often supplementing the jets. Electric current passing through loops of wire mounted in the spacecraft would develop magnetic fields which, reacting against the magnetic field of the earth, would exert a torque on the satellite that could be used to alter the orientation. Rapidly spinning wheels -gyroscopes-that stubbornly resist efforts to change their orientation in space, could also help point and stabilize spacecraft. Most of the structure of the Orbiting Solar Observatory rotated to provide gyroscopic stabilization. Finally, if the mass of a spacecraft was distributed much as the material in a dumbbell, the earth’s gravity could keep the satellite pointed toward the earth’s surface, as in Applications Technology Satellites. Since the earth’s gravitational field varies inversely with the square of the distance from the center of the earth, the end of such a spacecraft nearer the earth would experience a greater pull of gravity than would the end farther away. Thus, whenever the end facing drift away, the greater force of gravity on it would pull that end back toward the earth again.
(MISSING PICTURES)
Explorer satellites. The great variety is impressive, but underlying that variety was a common technology.
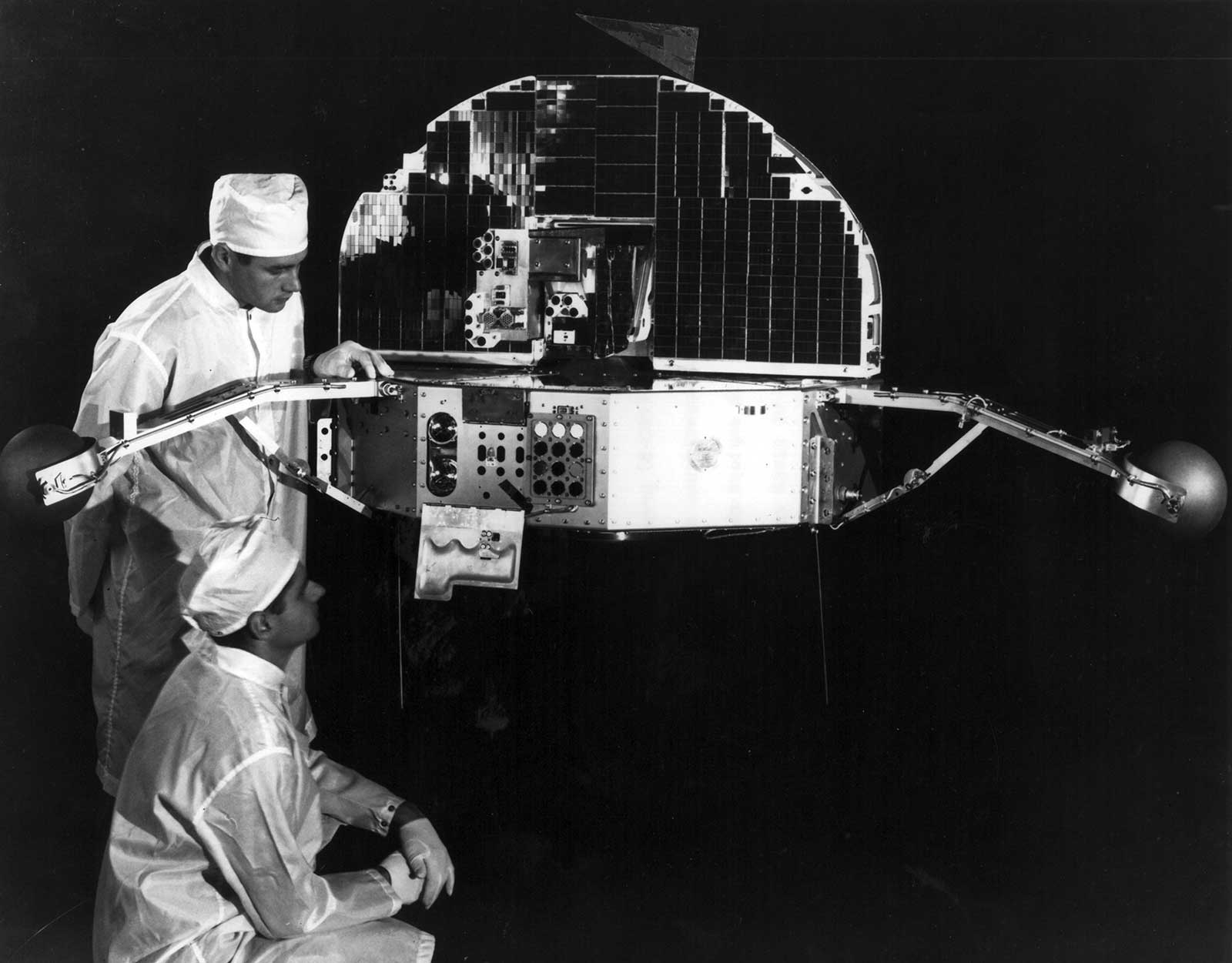
Orbiting Solar Observatory. OSO was the first observatory-class satellite built by NASA. In the photo above (fig.16), OSO 3 is prepared for tests before launch into orbit in 1967.
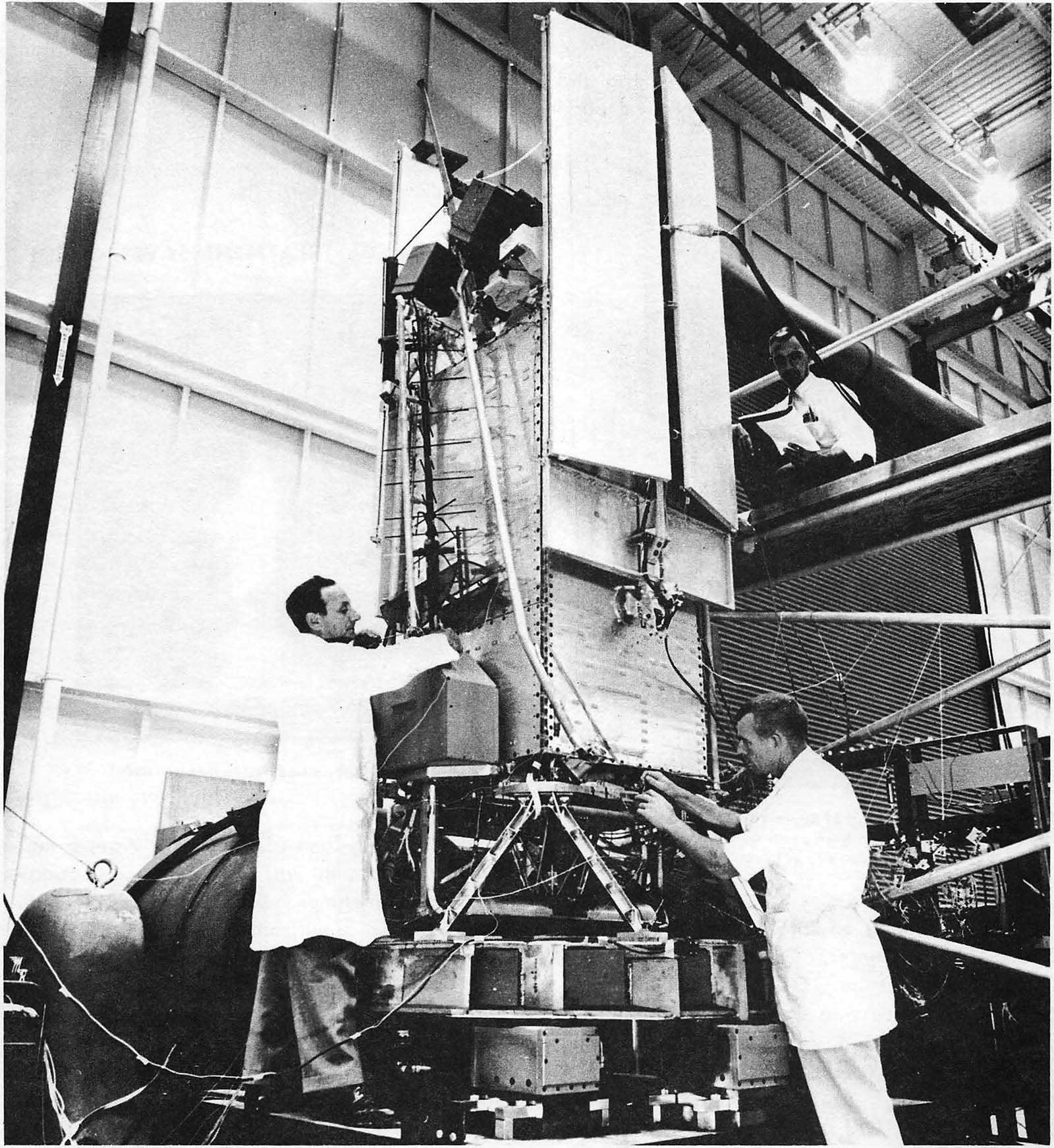
Orbiting Geophysical Observatory, a very capable spacecraft. Although once dubbed the “streetcar satellite,” OGO never acquired the ease of preparation hoped for it. OGO 4’s booms, antennas, and solar panels are folded in the photo above, for vacuum chamber tests and launch in 1967. OSO was the first observatory-class satellite built by NASA. In the photo above (fig.17), OSO 3 is prepared for tests before launch into orbit in 1967.
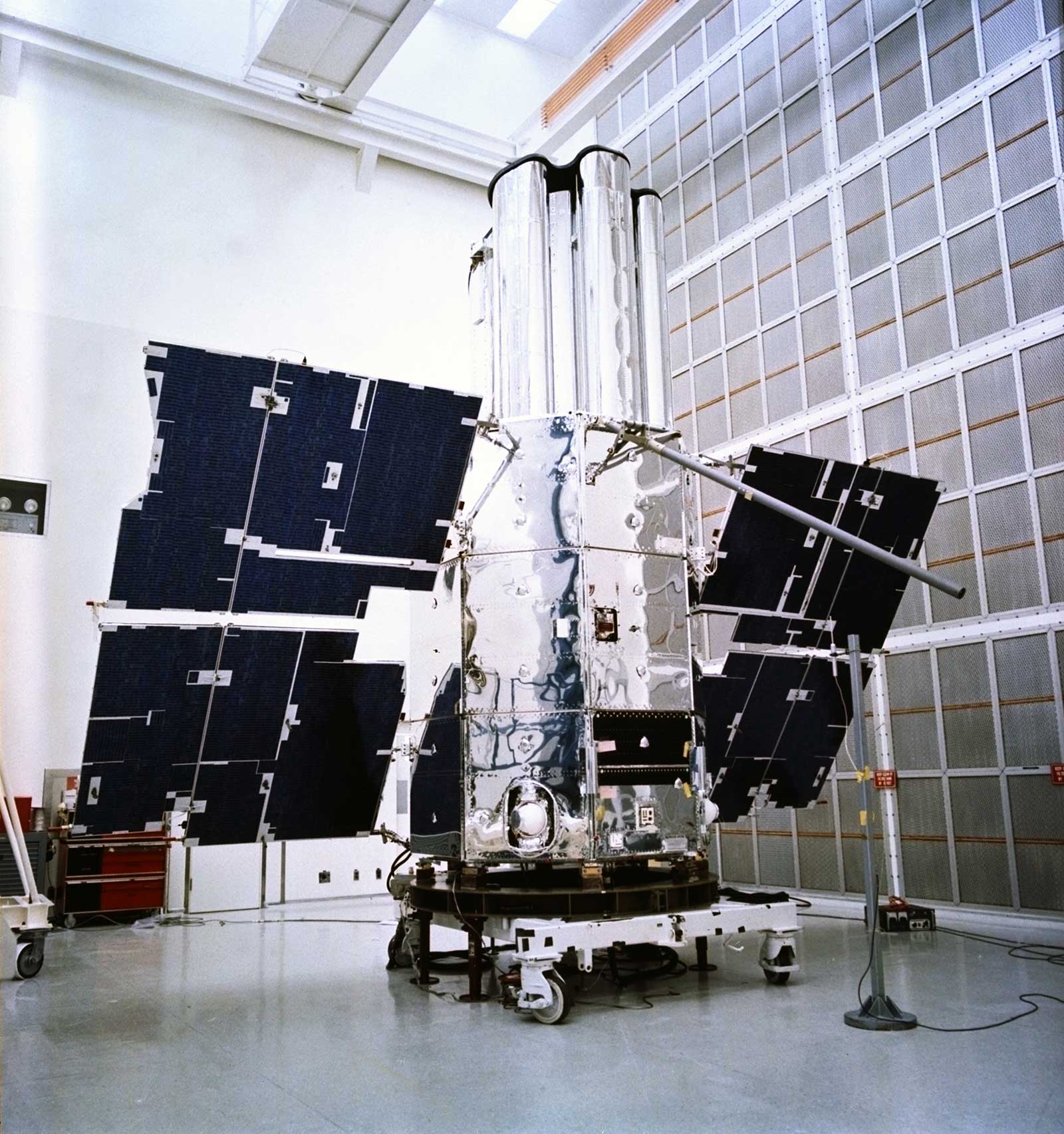
Orbiting Astronomical Observatory. OAO was the most complex and difficult of the observatory-class satellites initially undertaken by NASA. Above (fig.18), solar panels are mounted on OAO 3 in Preparation for its 1972 launch.
Observatory-size spacecraft were also large enough to carry additional rockets which, when fired against the direction of the satellite’s motion, could return the spacecraft to earth, where its equipment and records could be recovered. Once on its way down, the spacecraft would have to be protected against heat generated by friction of the atmosphere, for which purpose retrorockets-that is, rockets fired in the direction of motion to slow the spacecraft-or parachutes, or a combination of the two, could be used.
Finally, there were the manned spacecraft (figs. 19-22). These were even larger than the unmanned observatories, since, in addition to housekeeping equipment and instruments, the spacecraft had to be completely maneuverable to return the astronaut to earth after the mission and also had to afford a suitable environment for the crew.
All three classes of satellites had their uses. The manned spacecraft introduced the element of exploration-of personal, on-the-spot investigation-into the program. With men aboard it became possible to extend laboratory research into the environment of space, as was done dramatically in Skylab and the Apollo-Soyuz Test Project.26 When Space Shuttle development was begun in the early 1970s, one motivation was to make it relatively easy for researchers to perform experiments in space laboratories.
At the other end of the spectrum, Explorer-class satellites permitted scientists to perform a wide range of space science experiments, as shown in table 5. To the scientists, the lower costs meant that more of the funds available could be put into the scientific research itself. Also, the effort required to put an experiment into an Explorer was considerably less than that required for an observatory-one or two years as opposed to many years. When a discovery was made, an experimenter could more quickly follow up with new experiments on sounding rockets and Explorers than he could using observatories. Moreover, not having to fit his instruments alongside those of many other experimenters-with all the problems of electrical, radio, and other kinds of interference-the experimenter could exercise greater control over his experiment. These advantages account for the unvarying insistence of the scientific community that NASA continue to provide sounding rockets and small satellites. Whenever larger projects appeared to threaten the funding of the smaller ones, the scientific community rose in defense of the smaller. Over this issue the scientists came as near to unanimity as they ever did.
On the other hand, some investigations required a greater capability than the Explorers afforded. Astronomy experiments that required large telescopes and precision pointing are one example. Bioscience experiments that required the recovery of specimens after exposure to the environment of space-as in the Biosatellite-are another.27 The Orbiting Geophysical Observatory afforded the means of conducting 20 or more experiments on one spacecraft, so that a variety of related phenomena could be observed simultaneously and correlated.
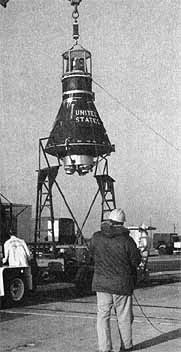
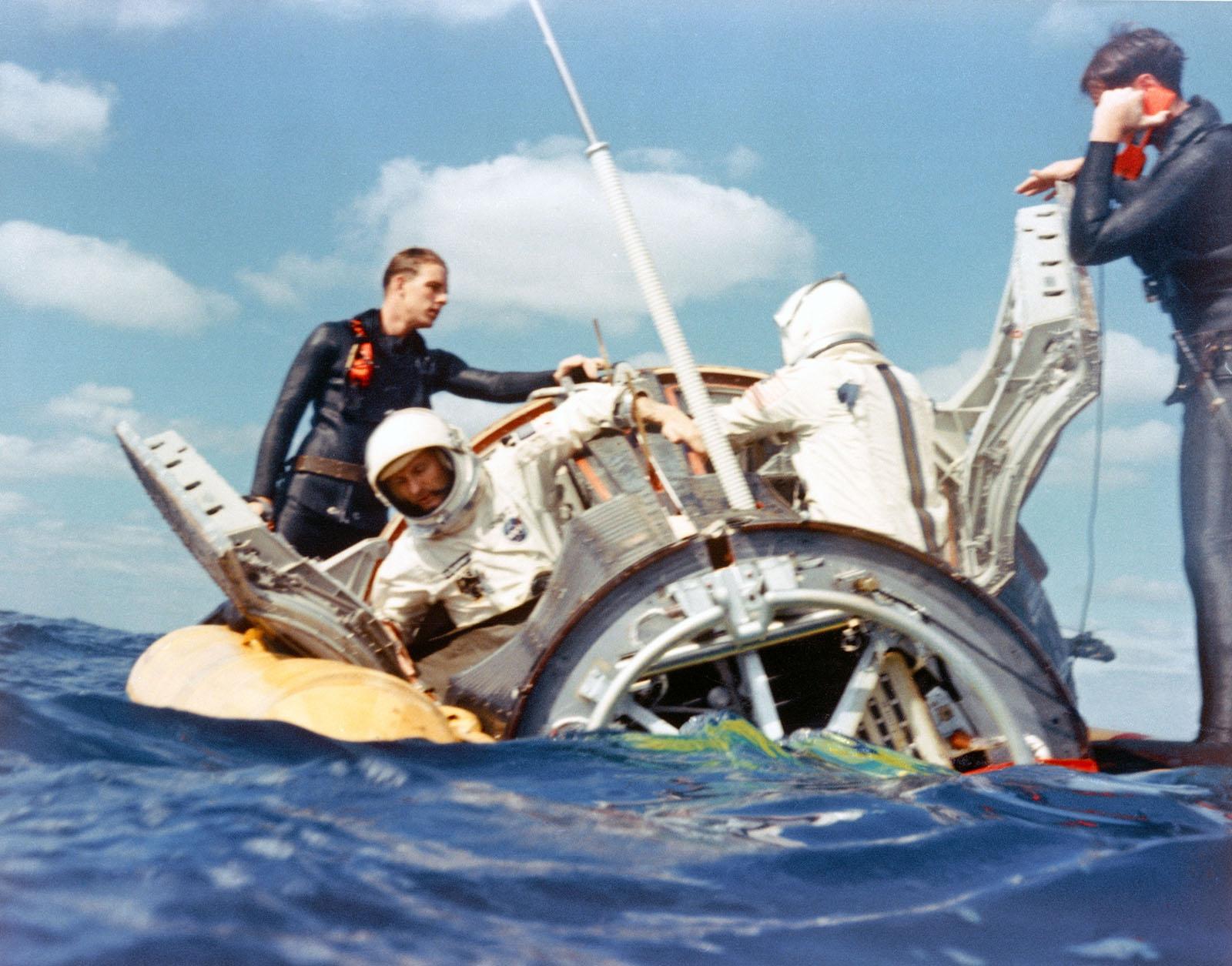
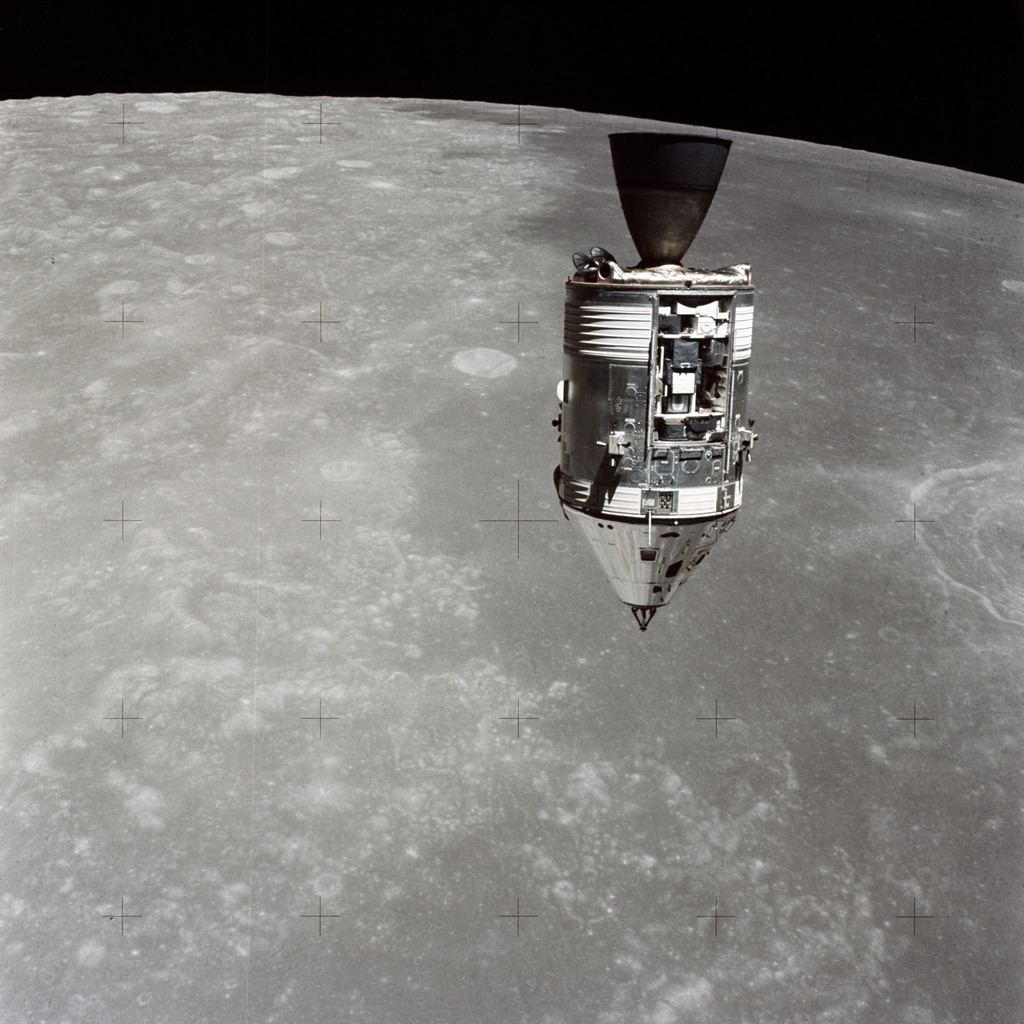
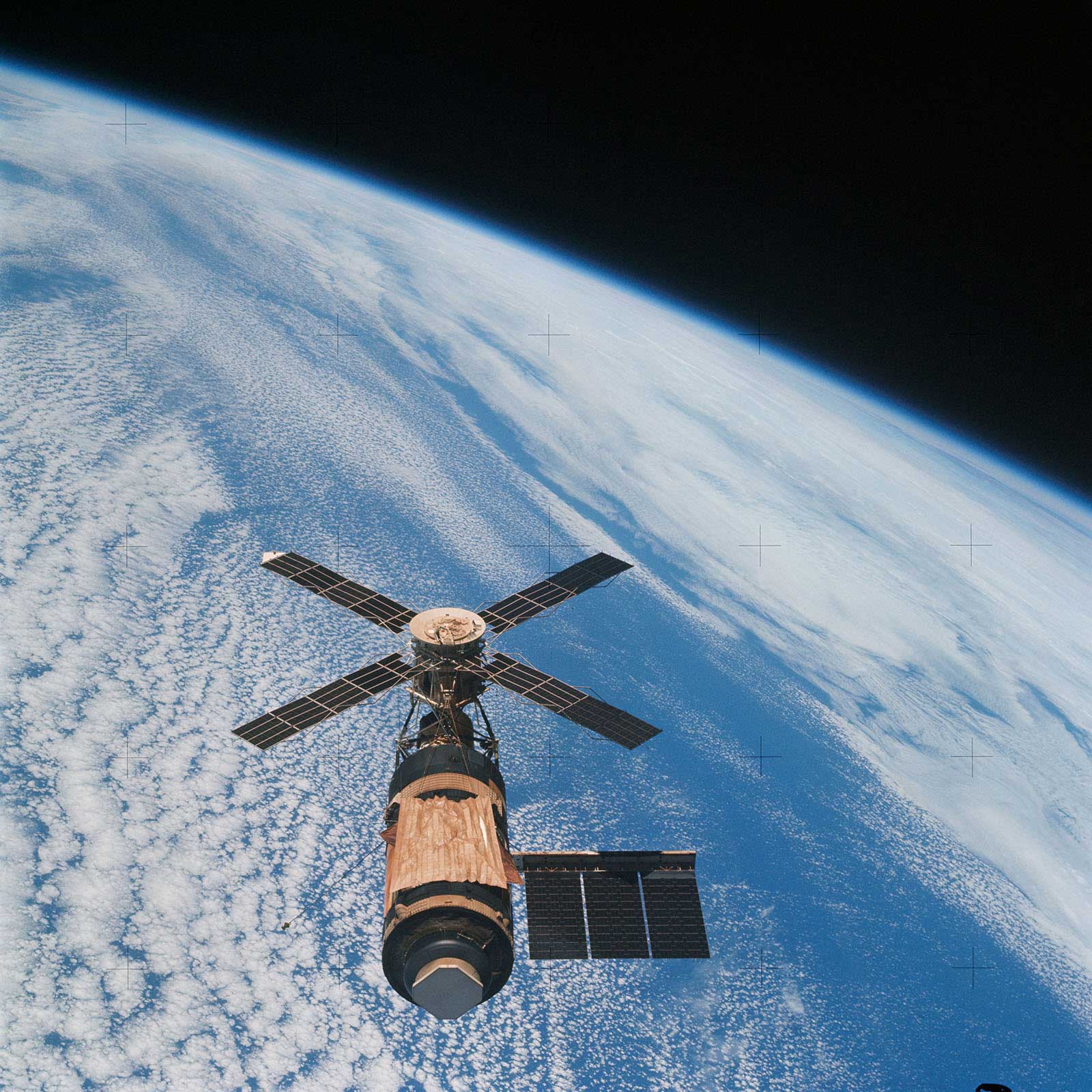
Manned spacecraft. Mercury (Figure 19) and Gemini (Figure 20) laid the groundwork for Apollo (Figure 21) while Skylab (Figure 22) grew out of the Apollo technology.
Examples of Investigations in Explorer-Class Missions
Spacecraft | Subject of Investigation |
---|---|
Vanguard 1 | Satellite geodesy. |
Vanguard 2 | Cloud-cover studies. |
Vanguard 3 | Survey of earth’s magnetic field and lower edge of radiation belts. |
Explorer 1 | Charged particle radiations in space. |
Explorer 6 | Radiation belt; meteorology. |
Explorer 7 | Energetic particles; micrometeoroids. |
Explorer 8 | Ionosphere; atmospheric composition. |
Explorer 9 | Atmospheric pressures and densities. |
Explorer 10 | Interplanetary magnetic field near earth; particle radiations. |
Explorer 11 | Gamma rays from space. |
Explorer 12 | Magnetospheric studies. |
Explorer 13 | Micrometeoroids. |
Explorer 14 | Charged particles and magnetic fields in magnetosphere. |
Explorer 16 | Micrometeoroids. |
Explorer 17 | Atmospheric composition. |
Explorer 18 | Charged particles and magnetic fields in cislunar space. |
Explorer 20 | Probe of topside of ionosphere. |
Explorer 27 | Geodesy by radio tracking methods. |
Explorer 30 | Solar x-ray studies. |
Explorer 38 | Radio astronomy. |
Explorer 42 | Catalog and study of celestial x-ray sources. |
Pioneer 5 | Interplanetary charged particles and magnetic fields. |
Ariel 1 | Ionospheric and solar research. |
Ariel 2 | Atmospheric research and radio astronomy. |
Alouette 1 | Charge densities in upper ionosphere and radiation belt studies. |
San Marco 1 | Atmospheric physics. |
ESRO 2B | Cosmic rays and radiations from sun. |
As with the launch vehicles, it proved impossible to find a single spacecraft carrier that would serve all needs, although some attempts were made in this direction. One spoke of building a standardized satellite, to effect economies and improve reliability. When conceived, the Orbiting Geophysical Observatory was described as a “streetcar satellite,” whose continuing use would so reduce the preparation time for an experiment that researchers could get their equipment aboard at the last minute-like catching a streetcar or bus-to follow up on some recent space science discovery. Initial reaction to the streetcar concept was positive, and the idea had the blessing of the Space Science Panel of the President’s Science Advisory Committee.28 But the problems of serving so many experimenters on one spacecraft defeated the objective. For each observatory a great deal of tailoring was required, compromises had to be worked out on orbits, orientation, placement of instruments, magnetic cleanliness of the spacecraft, allocation of telemetering capacity, and operating time. Use of a common electrical power supply invited electrical interference among different experiments, and often an offending experimenter was required to provide his own power. Ionospheric and radiation belt phenomena were fundamentally related to the earth’s magnetic field, making it important to measure ions, radiation particles, and magnetic fields simultaneously. But the various measuring instruments could easily interfere with each other unless care was taken. Those who wished to determine atmospheric composition at spacecraft altitudes required that the satellite and other instruments not contaminate the natural atmosphere with gases brought up from the ground-another difficult problem when large numbers of experiments were being conducted simultaneously.
Such problems defeated the efforts to produce standardized satellites in the same sense as standardized autos and auto parts or standardized home appliances. Nevertheless a considerable amount of uniformity was achieved. The basic structure, housekeeping, and orientation systems of the solar observatories were essentially the same from spacecraft to spacecraft. Even the geophysical observatories, with all the tailoring that they required, had much in common with each other. More important, the technology on which spacecraft were based acquired over the years a certain amount of standardization.
In this sense even the Explorers were standardized. Certainly no more varied looking group of satellites could be assembled than those of figure 15, which shows a large number of the Explorer satellites. Yet they were all cousins, stemming from a common, rather straightforward technology. When an engineer started out to design an Explorer-class satellite, he had pretty much in mind the kinds of structure, temperature control, tracking and telemetering devices, and antenna systems he might use. He was familiar with the kinds of vacuum, thermal, and vibration tests the spacecraft would have to pass to be approved for flight. To be sure, the technology advanced over the years as better components and materials became available, and improved housekeeping equipment was devised. But the family relationship remained. The steady, though gradual, change in Explorer technology did make the later Explorers considerably more capable than earlier ones. For example, Explorer 35 launched on 19 July 1967, weighing 104 kg and operating from an orbit of the moon, could far outperform the first several Explorers which weighed only tens of kilograms. Yet in the evolution of the series, any given Explorer was quite similar in its technology to the immediately preceding one. The same point is illustrated by the solar observatories. These also changed gradually over the years. In August 1969 the sixth solar observatory, weighing 290 kg, went into orbit. Though looking a great deal like the first observatory, launched on 7 March 1962 and weighing 200 kg, OSO 6 was more versatile than earlier ones, having the capability to point two telescopes at the sun to study in detail ultraviolet and x-ray spectra at any point on the solar disk.
Like the earth satellites, space probes-which were spacecraft sent away from earth into deep space-fall into several classes. The analogy is very close. Akin to the Explorer satellites were the Pioneer space probes (fig. 23). These were modest-sized vehicles, ranging from around 40 kg in the first models to the 260-kg weights of Pioneer 10 and 11 sent to Jupiter in 1972 and 1973. They were spin-stabilized and instrumented to investigate the interplanetary medium and the environs of a planet as the spacecraft flew by. During the 1960s a number of Pioneers revolving like little planets about the sun provided a great deal of information on the solar wind and magnetic fields in space. When on the far side of the sun from the earth, Pioneer radio signals were carefully observed to find effects of the sun’s gravity predicted by the general theory of relativity. On 3 December 1973 Pioneer 10 passed Jupiter at 130,000 km from the planet’s surface-taking pictures, measuring its radiations, and investigating some of its satellites-and then receded along a trajectory that would eventually carry it out of the solar system. A year later Pioneer 11 also flew by Jupiter, sending back more data on the giant planet and its satellites, leaving the planet on a course that would carry the spacecraft in September 1979 to the vicinity of the second largest planet, Saturn, famed for its rings. These missions into deep space give some idea of Pioneer’s usefulness to the space scientist. As with Explorer, Pioneer technology also advanced steadily through the years, so that in the early 1970s it was possible to plan to use Pioneer spacecraft to carry orbiters and atmospheric probes to Venus in the late 1970s.29
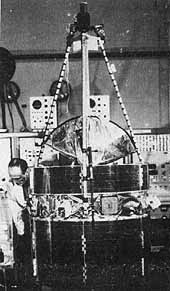
Pioneer. The earliest of the U.S. space probes provided the kind of flexibility for the deep-space investigator that Explorers provided for earth-satellite missions. Pioneer 8 in the photo is prepared for December 1967 launch into orbit of the sun.
Analogous to the observatory satellites were the larger lunar and planetary probes: Ranger, Surveyor, Lunar Orbiter, Mariner, and Viking (figs. 24-28). Like the observatories, these spacecraft were maneuverable and, using a celestial reference system, could be accurately oriented in space. In addition to small jets for orienting the spacecraft, they also carried a larger rocket that could be fired to alter the trajectory so as to keep the craft on course to its intended target.
Lunar Orbiter carried enough auxiliary propulsion to place it in a lunar orbit, from which the spacecraft obtained a series of photographs of the moon. These were later used to produce maps of the moon’s surface and to aid in planning Apollo missions. Several Mariners carried enough propulsion to place them in orbit about Mars. Surveyor used retrorockets to slow the spacecraft for a soft landing on the moon, after which remotely controlled instruments televised and investigated the surrounding landscape. Viking combined both the orbiting and landing capability the main vehicle first going into orbit of Mars, after which a portion of the spacecraft separated and was forced by rockets to descend to the Martian surface.
Deep-space probes had to overcome problems additional to those encountered by earth satellites. For example, a Martian probe took about two-thirds of a year to get to its destination. Pioneer 10 and 11 required almost two years to fly to Jupiter, and had to survive those two years in the environment of space to accomplish their assigned missions. If an earth satellite operated properly for a few months, the experimenters would have a few months worth of data for their trouble; but if a planetary probe operated for only a few months, they would get no planetary data at all. Of course, one also made interplanetary measurements on planetary flights-observing the solar wind, magnetic fields in space, dust, meteor streams and cosmic rays-but on planetary missions these were secondary objectives.
Another requirement for the great distances traveled by interplanetary and planetary spacecraft was more powerful radio communications systems. The antenna had to be pointed toward the earth. If omnidirectional or wide-angle antennas were used, the power requirements went up, sometimes prohibitively. If, to conserve power, narrow-beam antennas were used, they exacerbated the antenna-pointing requirement. Finally, spacecraft that flew toward the sun had to be protected against overheating by solar radiations, while those that flew away toward the outer solar system had to be protected against freezing.
With these larger space probes one could plan in the course of time to investigate all the planets and major satellites of the solar system, and the asteroids and comets. Spacecraft could be placed in orbit around other bodies, as was done with Lunar Orbiter around the moon and Mariner around Mars. Landers could place instrumented laboratories on the surfaces of other bodies, as did Surveyor and the Soviet Luna on the moon, Viking on Mars, and Soviet Venus probes on Venus. It was even possible to deposit roving laboratories on those bodies, as the Soviet Union did with Lunokhod on the moon, or retrieve samples of material from them as did Luna 16 and 17. 30
Finally, there were the manned space probes (fig. 29). During the 1960s these consisted solely of the Apollo-Lunar Module combinations that the American astronauts flew to the moon. As with the manned satellites, these provided the added dimension of manned exploration. The successful Apollo missions yielded such a wealth of scientific data as to soften at last the years-long lament of the scientific community over the tremendous expense of the Apollo program.
Of course, operation of these spacecraft required auxiliary equipment and systems. Out of the Minitrack tracking and telemetering network of the International Geophysical Year grew a versatile satellite network for issuing instructions to satellites, determining their orbits, and receiving telemetered information.31 To work with the deep-space probes, the Jet Propulsion Laboratory established a deep-space network using 26-m and 64-m parabolic antennas at three stations spaced roughly equally around the world in longitude, so that a distant space probe could at all times be viewed from at least one of the stations.32 For manned spaceflight a special network was linked to the Johnson Space Center in Houston.33 As needed these were supported by tracking-telemetering ships and aircraft furnished by the Navy and the Air Force.
The rockets and spacecraft were a sine qua non of the space program and of space science. It is not surprising, therefore, that most of NASA’s activity and resources went into the creation and operation of these vehicles. The scientific researches themselves, while not inexpensive, required only a fraction of what the tools-the spacecraft and launch vehicles-cost. Since the tools were where most of the money was going, Congress spent a great deal of time probing the budgets for them, and NASA managers became accustomed to thinking of their programs in terms of launch vehicles and spacecraft. One would speak of Ranger and Mariner programs, and of the Polar Orbiting Geophysical Observatory program and the Orbiting Solar Observatory program-or rather, to the distress of those to whom acronyms are anathema, of the POGO and OSO programs. From time to time scientists would chide NASA on this habit, pointing out that as far as space science was concerned, the program was to investigate the magnetosphere, to probe the origin and evolution of the moon and planets, to understand the solar processes, etc. NASA managers agreed, of course, and indeed from the very outset NASA people set forth the scientific objectives, not the space hardware, as the purpose of the space science program.34 But the shorthand was too convenient, and the practice persisted even among the scientists.
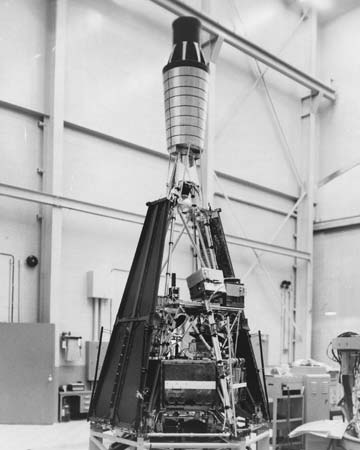
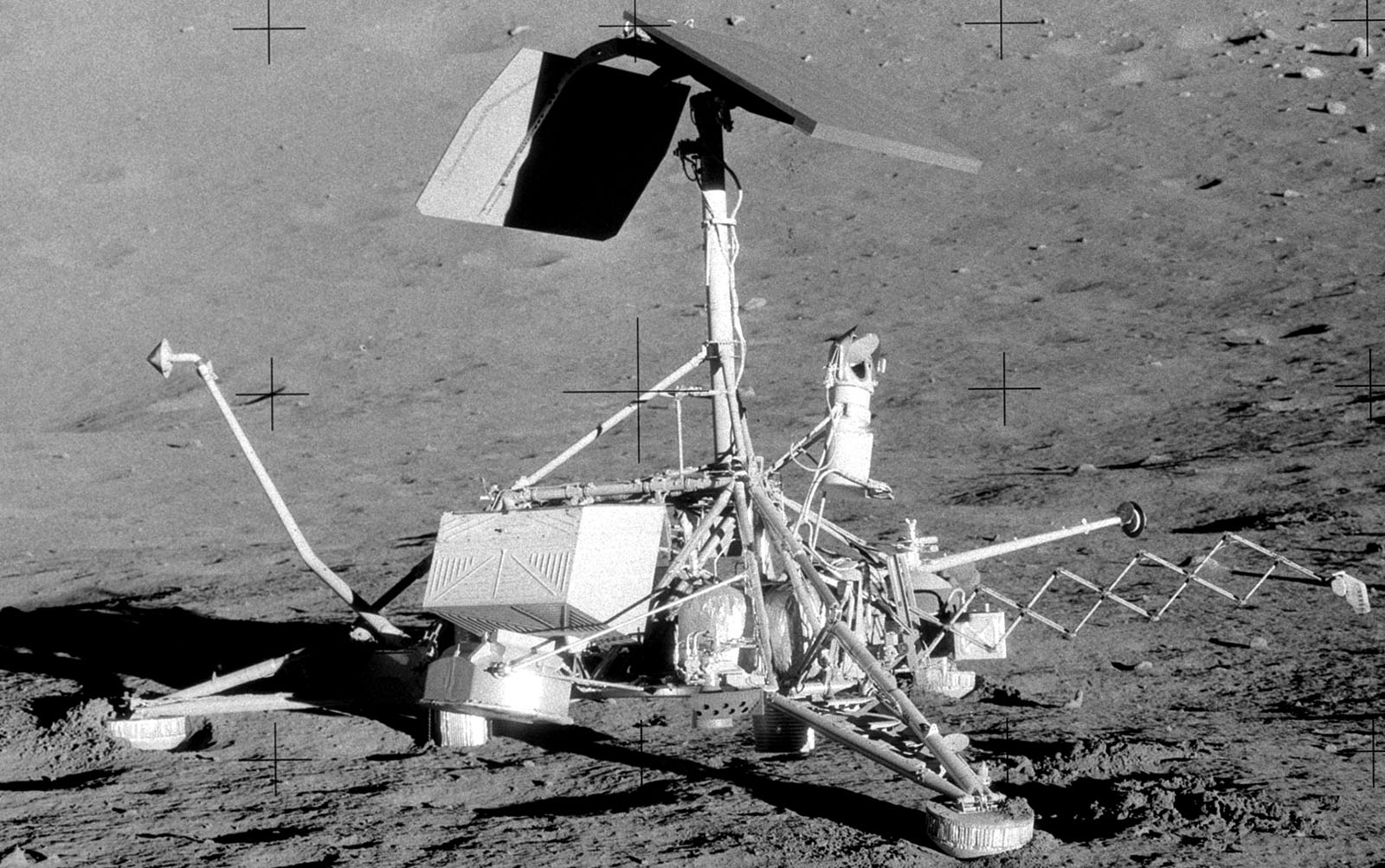
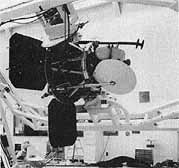
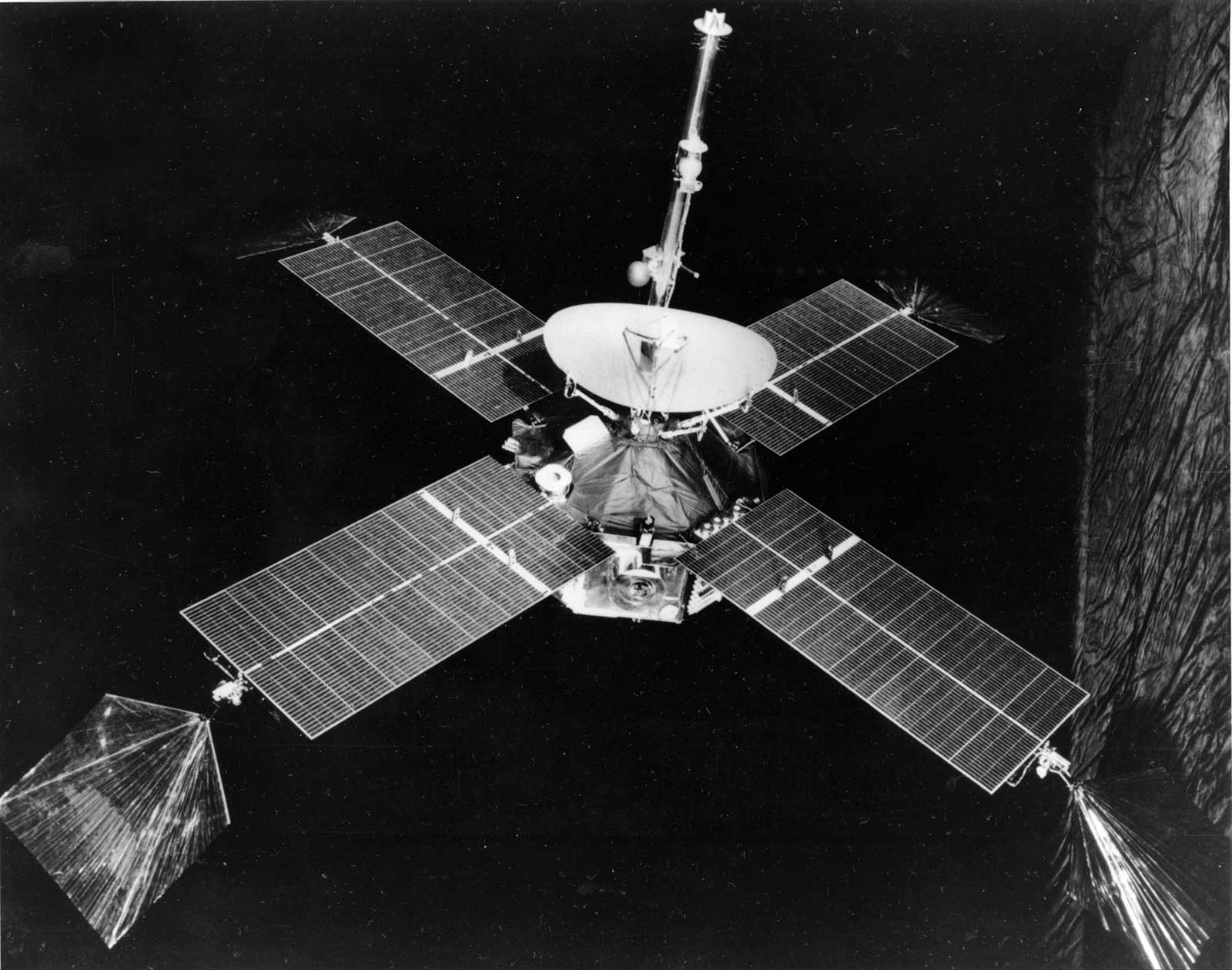
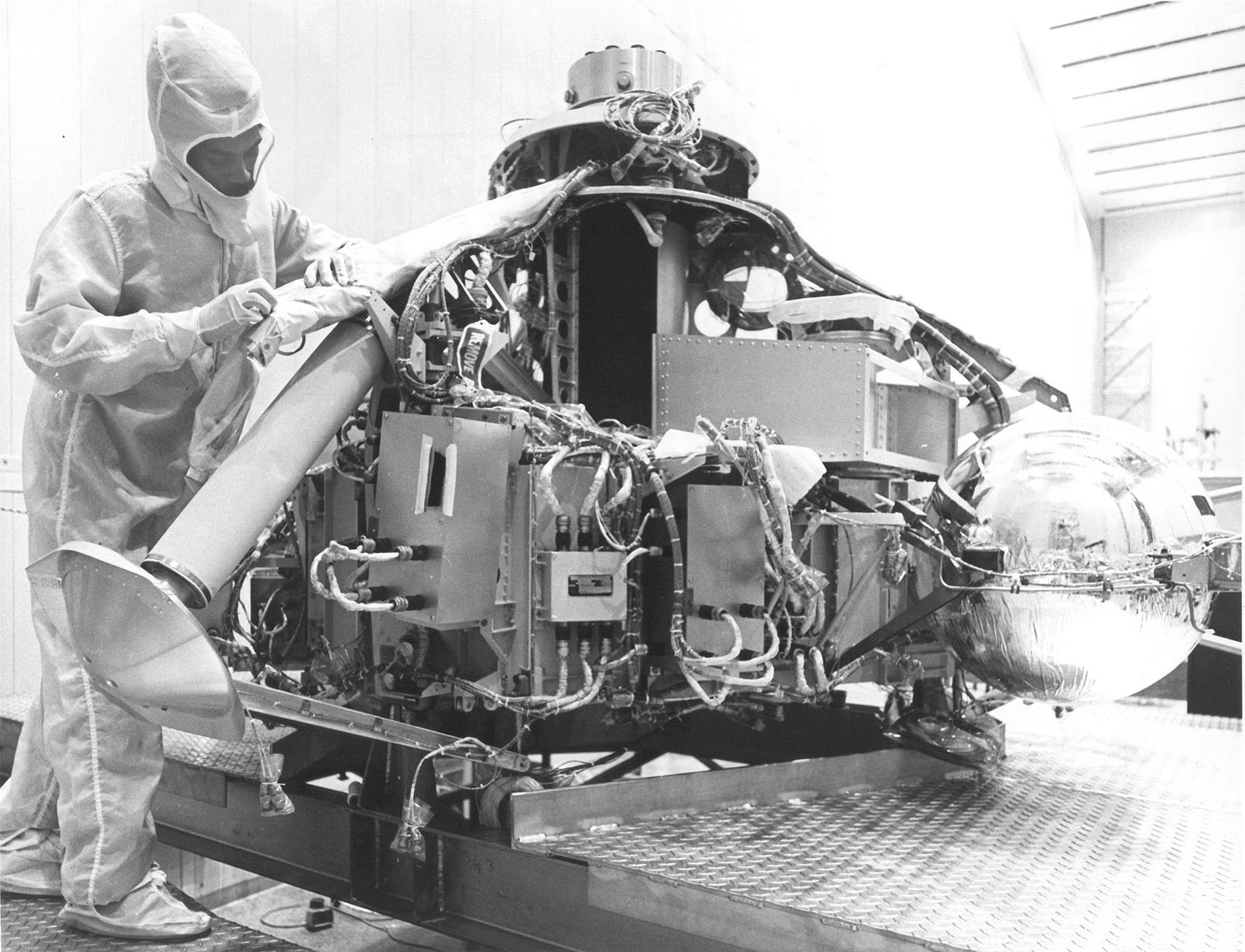
Lunar and planetary probes. These were the deep-space analogs of the observatory-class satellites: Ranger, (Figure 24); Surveyor, (Figure 25), on the lunar surface; Lunar Orbiter, (Figure 26); Mariner (Figure 27); Viking (Figure 28)
HARD-LEARNED LESSONS
The rockets and spacecraft that won NASA an image of success did not come easily at first. During NASA’s first two years the launch vehicles especially produced their share of grief. There were important lessons to learn, and experience proved to be a stern teacher. Atlas-Able and Pioneer provide a good illustration.
The Atlas-Able-a launch vehicle using the Atlas missile as the first stage combined with a second stage from the International Geophysical Year’s Vanguard-had been brought into the spaceflight program by Abe Silverstein, head of NASA’s Office of Space Flight Development. NASA hoped to fill in and perhaps even to steal a march on the Soviet Union in lunar and planetary exploration while the agency pressed the development of its own space launch capability.35 Contracting with Space Technology Laboratories for three deep-space probes-Pioneer spacecraft-to be launched by a space vehicle yet to be proved, NASA hoped to move faster in its science program than would otherwise be possible with the smaller rockets available to the agency. At first intended to launch a Venus probe, Atlas-Able was switched to lunar missions when the planetary flight appeared to be too much to attempt at the start. Hopes ran high for Atlas-Able and a number of scientists vied to help outfit the probe with scientific instruments. They had reason to be unhappy when their hard work went for naught.
Pioneer spacecraft on the Atlas-Able vehicles carried instruments to investigate interplanetary space and the moon. The Pioneer program, begun by the Air Force, was taken over by NASA when the new agency assumed responsibility for the nation’s space science program.36 Between 11 October 1958 and 15 December 1960, eight attempts were made to launch Pioneers into space.37 Six, including all three Atlas-Able firings, were failures. Pioneer 4, riding a Juno II launch vehicle, achieved a limited success.
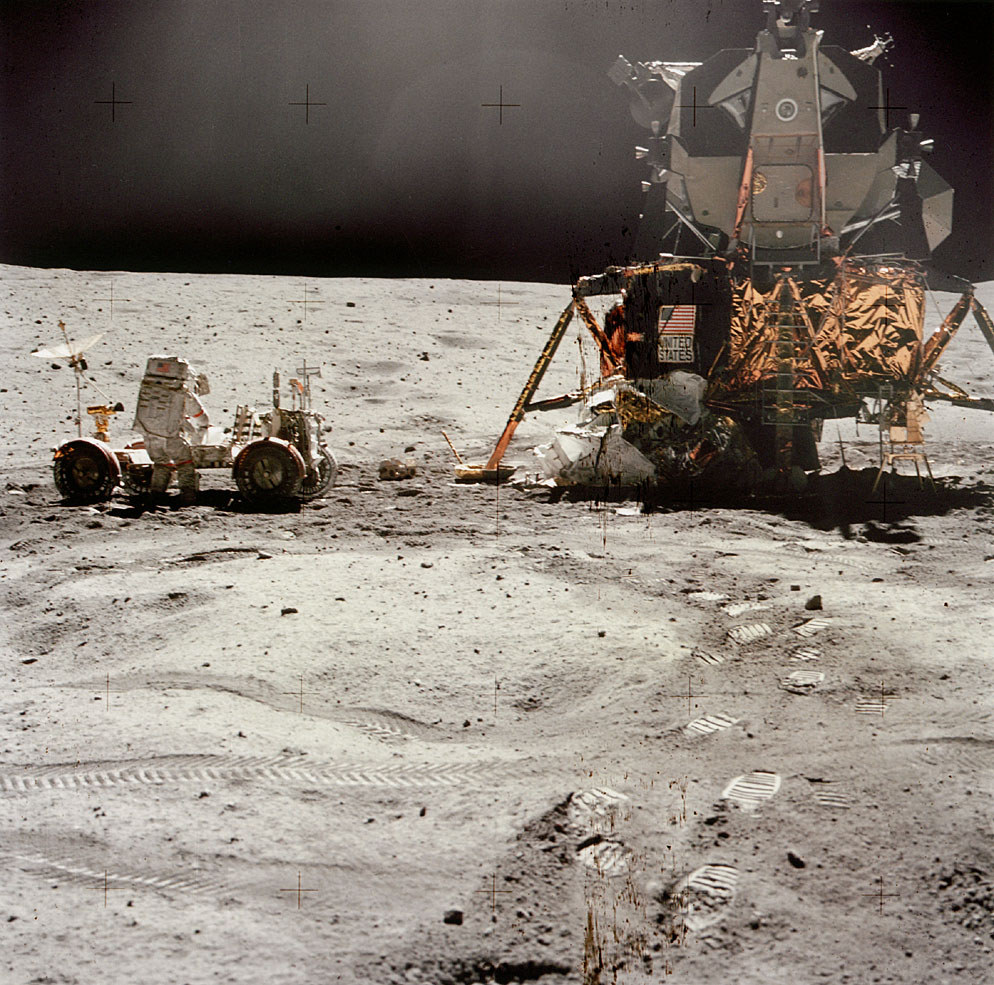
Apollo lunar module. Six LMs descended from lunar orbit to land men on the moon, three of the craft carrying a lunar roving vehicle. Above, Apollo 16 LM and Rover on the moon in 1972 (with lunar dust on the camera lens showing in streaks.
Only Pioneer 5, launched by a Thor-Able on 11 March 1960, could be called an unqualified success. Although instruments on Pioneer 5 provided a great deal of information on cosmic rays and the space environment, the success could hardly erase the gloomy picture of six outright failures.
The significance of the Pioneer and Atlas-Able failures was all too clear at the time. In retrospect that significance stands out in high relief. For scientists it emphasized the sad plight that could await the experimenter who put his instruments on an as yet unproved vehicle. Long months, even years, of hard work could prove fruitless if the vehicle did not do its job properly. John Simpson of the Fermi Institute at the University of Chicago had reason to lament this hard fact of life. His group had developed new instruments to investigate the composition and energy of the solar wind and had accepted an invitation to put their instruments aboard the Pioneers, of which only Pioneer 5 had been satisfactory.
The fiasco precipitated a long series of exchanges between Simpson and NASA in which the frustrated investigator explored ways of recouping his losses by gaining access to other NASA flights. On 16 December 1960, the day after the last Atlas-Able attempt, Simpson was on the phone urgently reviewing his situation with the author.38 A half year later Simpson wrote that his group had participated in at least eight launchings with only two successes, a circumstance he attributed to having the Chicago experiments flown on unproved rocket systems or being assigned the role of secondary objectives.39
NASA had to face the issue of backups for important experiments, an issue that would always be at the back of an experimenter’s mind when he signed up for a long difficult period of preparing for a space mission.40 It soon became apparent that the time and effort required to conduct an experiment on a satellite or a space probe was far greater than that required to perform experiments in the laboratory. For the laboratory, one normally thought in terms of months, whereas space experiments could require years of hard work. Scientists began to point out that taking part in one or a few space science experiments could consume an appreciable fraction of a person’s productive career, and in a “publish or perish" world the failure to get results because a rocket or a spacecraft didn’t function properly could seriously affect that career. NASA was early moved by such considerations to adopt a policy of rescheduling experiments which, through no fault of the experimenter, did not succeed.41
Atlas-Able made plain that success on space missions would be neither automatic nor cheap. There was a price to pay, and part of that price was failure of some missions. This price NASA management would never find comfortable. The trouble with a philosophy of accepting a certain number of failures as normal and inevitable, was that even “learning failures" in an open program like NASA’s, conducted under the watchful eye of a whole world, looked to the public and Congress like absolute failures. The press treated them as failures. It didn’t matter that previous development projects like the V-2, Atlas, Thor, Polaris, and almost any other major rocket development one could name, had had their share of unsuccessful early firings, and that this had been accepted as a necessary growing pain. Those difficulties had been hardly visible under the cloak of military secrecy. But space program difficulties were highly visible and distressing.
The first notable application of a double standard came even before NASA was formed, with the spectacular Vanguard explosion in December 1957.42 Before the Vanguard development ever got under way, it had been agreed at the National Academy of Sciences that if only one International Geophysical Year satellite out of six made it to orbit, that would be taken as a successful outcome of the project, so difficult was it considered. In fact, when the number of launches for the program was cut back from 12 to 6, the scientists argued strongly that they couldn’t reasonably expect more than two successful flights.43 Actually three were successfully launched before the end of the program. Moreover, out of the Vanguard development came the liquid- and solid-fueled upper stages that made the Delta launch vehicle the tremendous success that it became. In addition, the Vanguard program contributed the Minitrack and Baker-Nunn tracking nets to help get the new space program off to a good start.
By any reasonably objective measures-certainly by previously accepted standards-Vanguard was a successful development. Yet the early launch failures made the entire program a symbol for failure in the public mind.
The lesson of Vanguard was plain. NASA could not afford to regard failure as acceptable under any guise. Success had to be sought on the first try, and every reasonable effort bent toward achieving that outcome. This philosophy was epitomized in the “all-up" approach adopted by George Mueller, who had taken over direction of NASA’s manned spaceflight programs in September 1963.44 In Apollo the all-up philosophy-which called for assembling a complete launcher and attempting to carry out a complete mission even on the early test flights-was intended to produce economies as well as to preserve an image of success.
But the all-up approach had already been applied to other NASA projects, particularly in the space science and applications areas. Explorer satellites were simply expected to succeed, and did. The Orbiting Solar Observatory was fully instrumented for space science on its first launch in March 1962. The flight to Venus in August 1962 was Mariner’s maiden voyage. Later, Associate Administrator Seamans did not permit the Office of Space Science and Applications to try for only a part of the project’s objectives on early launches of Surveyor to the moon. He insisted on a full-scale mission on the first lunar flight attempt. It became customary to plan for only one or two spacecraft for a project, expecting those to meet the desired objectives. While this policy kept the question of backups always in view, and was a source of some uneasiness, the detailed attention required to make the policy of success-on-the-first-try work was important in building confidence in NASA’s ability to do its job.
And that was the third issue highlighted by the ill-fated Atlas-Able project. While getting on with the business of systematically building up the national launch capability, NASA had more or less turned over the handling of Atlas-Able flights to the industrial contractor. It was not a procedure that the agency would follow very often. Rather, with its open program-operating in a goldfish bowl, as it were-the agency would prefer to monitor its contractors very-closely, often more closely than the contractors thought necessary. Indeed, a great deal of NASA’s management time would be taken up in overseeing the work of contractors on rockets, spacecraft, and other equipment needed for the space program.
Finally, the Atlas-Able missions served to emphasize the key role played by the rocket in space operations. Successful rockets opened up space to human exploration. Without rockets, space must remain ever remote and inaccessible. Moreover-and this was the point behind the Atlas-Able attempts-some rockets could do more than others. Neither the Jupiter C, which had launched Explorer 1, nor the Vanguard launch vehicle could send probes to the moon or planets. With such vehicles one would have been restricted essentially to small artificial satellites of the earth. The limitations of these first American launch vehicles were further emphasized by comparison of the 8-kg Explorer 1 and the 28-kg Vanguard 3 with the 84- and 508-kg weights of Sputnik 1 and 2.45 Indeed, even more disturbing than the Soviet Union’s launching of the first satellite was its obvious superiority in launch vehicles and implied superiority in long-range missile capabilities. The disparity was brought out even further by the launch of Sputnik 3, weighing 1327 kg, on 15 May 1958.46
COSTS
Building the launch vehicles and spacecraft described in previous sections was expensive, but necessary if the United States was to achieve the stated objectives in science, applications, and exploration. Moreover, costs did not end with development. Since most rockets and spacecraft of the 1960s were expended in the accomplishment of their missions, it was necessary to buy a new launch vehicle and a new spacecraft for each new mission.
Individual costs for launch vehicles are given in table 6. These are rough, order-of-magnitude figures. Actual costs varied, depending on how many special requirements were placed on the launch by the science objectives. To start, launch vehicle costs exceeded those of the payloads they carried, but in just a couple of years Hugh Dryden was informing the Congress that spacecraft costs had come to exceed those of the launch vehicles.47 As time went on, engineers and scientists became expert in miniaturizing equipment, cramming their satellites and space probes with instruments. This practice increased the amount of research that could be accomplished in a given payload weight and space, but it also made for expensive spacecraft. From experience with Explorers, a rule of thumb developed that scientific spacecraft would cost about $20,000 to $40,000 per kilogram, but in time more complicated and sophisticated vehicles, such as the larger deep-space probes, were far more expensive. Typical costs for NASA scientific spacecraft are shown in table 7.
Vehicle | Cost of Hardware plus Launching* (millions of 1970 dollars) |
---|---|
Scout | 3.6 |
Delta (= Thor-Delta) | 9 |
Atlas-Agena | 17.8 |
Atlas-Centaur | 19.4 |
Titan IIIC | 28.6 |
Saturn IB | 56 |
Saturn V | 225 |
* These are only order-of-magnitude costs. Moreover, they are the prices after many years experience. NASA had hoped Scout would cost about $1 million per rocket, but both inflation and programs to improve performance raised the price substantially above the target. Inflation and improvement programs also increased the cost of Delta over the years.
The tabulation illustrates why many scientists were wary about getting into projects using the larger spacecraft. The cost of about three-quarters of a million dollars for four Vikings could pay for at least twice as many Pioneers, or for dozens of Explorers. When the costs of the Viking program continued year after year to delay undertaking Pioneer missions to Venus, there were strong protests. For both satellites and space probes, the larger spacecraft were recognized as essential to the accomplishment of many important investigations, but generally were not acceptable at the sacrifice of the smaller missions, which gave the scientist more flexibility and personal freedom of action.
Manned spacecraft were an order of magnitude more expensive that unmanned satellites and probes. This was so not only because of the larger size and greater complexity of vehicles that were to carry men, but also because every effort had to be bent to guarantee the safety of the crew. Trying to guarantee perfect performance was very costly, often requiring much redundancy. For unmanned spacecraft and launch vehicles, it did not make economic sense to try to achieve the same degree of refinement. Scout, for example, was designed to be an inexpensive launch vehicle for science and applications missions. Reasonable care and good engineering and operational practices could achieve success rates of 90 percent or better, and still keep the vehicle in the inexpensive category. To try to guarantee 100-percent success, on the other hand, would have increased costs enormously. Thus, if one required 10 successful firings for a certain program, and was willing to shoot for getting those 10 successes out of a total of 11 or 12 firings, the total program costs would be much lower than if one insisted on achieving the 10 successes with only 10 firings.
Spacecraft | Cost per Kilogram * (thousands of 1970 dollars) |
Explorer | 20-40 |
Interplanetary Monitoring Platform | 50 |
Orbiting Solar Observatory (depending on complexity) | 30-70 |
Orbiting Geophysical Observatory | 70 |
Orbiting Astronomical Observatory | 55 |
Small Astronomy Satellite | 174 |
High Energy Astronomical Observatory | 60 |
Surveyor | 85-90 |
Lunar Orbiter | 95 |
Pioneer (from no.6 on) | 175-225 |
Mariner | 85-105 |
Viking Orbiter | 85 |
* Order-of-magnitude figures only.
The author vividly remembers a discussion of this point before the Space Science and Applications Subcommittee of the House Committee on Science and Astronautics during hearings on NASA’s fiscal 1966 budget request. Concerned that NASA might be escalating the costs of Scout by insisting on too high a degree of reliability, Congressman Weston Vivian, himself a former engineer, queried Edgar Cortright, deputy in the Office of Space Science and Applications, on the matter.48 While acknowledging the desirability of striking a proper balance between costs and reliability, NASA people took special delight in this new twist. The normal experience was to be challenged to explain why the unmanned program wasn’t striving all-out, as in the manned program, to achieve perfection.
Neither NASA nor the Department of Defense had carte blanche to spend unlimited sums on rockets and spacecraft. While desiring that the nation’s space program should be first rate and that the country should regain the image of leadership in the field, the administration and Congress still were concerned that costs be kept down. While it was apparent at the outset that in time many agencies would come to be interested in applying space techniques to their work, it soon became equally apparent that these agencies could not expect to operate their own launch and spacecraft facilities. If the Weather Bureau, the Geological Survey, the Federal Aviation Administration, the Maritime Commission, the Department of Agriculture, and the Forest Service had attempted to run their own space programs, the aggregated costs would have been prohibitive. As a consequence it was expected that NASA, and occasionally the Department of Defense, would service other agencies wishing to use space methods in their own programs. As the number of space applications grew over the years, more and more of NASA’s work was expected to go into providing support to others.
But manpower and money were not the only price to pay for exploring and utilizing space. As the example of the ill-fated Atlas-Able missions showed, there were failures and frustrations to endure. Also, rockets and spacecraft could at times be hazardous. Perhaps the best known illustrations were the Apollo fire, in which three astronauts were burned to death in a tragic holocaust of flammable materials in the oxygen atmosphere used in the Apollo capsule, and the April 1967 flight of Soyuz 1, in which Cosmonaut Vladimir Komarov was killed.49 Because of the universal interest in the manned flight program, these tragedies received worldwide attention. Apollo, for example, became the subject of an intense, deeply probing congressional investigation.50 But others also gave their lives in the course of the program, though with less notice from the public. Astronauts who died in accidents in their training airplanes received only momentary notice. On 5 October 1967, at Northern American Rockwell’s plant in Downey, California, a hazardous mixture containing barium used in NASA sounding rocket experiments exploded, killing 2 workmen and injuring 11. The accident was thoroughly investigated by a NASA board and the procedures for handling such chemicals were revised. To the public, however, the matter appeared to pass as just another industrial accident.51
Just as tragic as the Apollo fire was the accident to an Orbiting Solar Observatory on 14 April 1964. In an assembly room at Cape Canaveral, Delta rocket’s third stage motor had just been mated to the spacecraft in preparation for some prelaunch tests. Suddenly the rocket ignited, filling the workroom with searing hot gases, burning 11 engineers and technicians, 3 of them fatally. An investigation following the accident showed that a spark of static electricity had probably set off the fuze that ignited the solid propellant.52 But, whereas the Apollo fire had evoked a national outcry, the OSO accident drew little attention except from those closely associated with the project.
One measure of the difficulty encountered in a development program was the increase in cost and schedules over the original estimates. When estimates proved on the mark, engineering difficulties had been correctly estimated and the project could be carried out in the specified time and for the stated price. But when unexpected technical problems required extra time to solve, costs increased and exceeded the original estimates. These overruns, as they were called, were usual in the complex, novel developments of the space program, and special management attention was needed
The Department of Defense had experienced such problems in the development and acquisition of large weapon systems. Studies showed that in the course of 12 major development projects, costs increased by an average 3.2 times and schedules lengthened by 36 percent.53 NASA fared little better.
Although the space agency, after its initial troubles, began to develop an enviable record of successes in its numerous programs, acquiring during the 1960s a reputation of being able to do what it set out to do, nevertheless the record was not as neat as the agency would have desired. An analysis in 1969 by D. D. Wyatt, who from the start had played an important role in NASA’s programming and budgeting, showed that cost increases over the life of a project were likely to rise substantially when the estimates were made before “establishing a well-defined spacecraft design and a clear definition of required experiment development.”54 Space science programs had their share of horrible examples. The Orbiting Astronomical Observatory, the Orbiting Geophysical Observatory, and Surveyor all increased in cost by about four times, as did the meteorological satellite, Nimbus. In contrast, the communications satellite projects Relay and Syncom, and the Applications Technology Satellite, for each of which a good definition of requirements was reached before estimating, showed only moderate cost increases, by between 1.1 and 1.3 times. While the manned spaceflight projects showed somewhat lower cost rises, Wyatt noted that the cost projections were made a considerable time after the projects had started and that there was evidence that estimates at the true start would have been much lower and cost increases accordingly much higher.
Wyatt’s basic thesis was correct. His analysis, however, did not go unchallenged. Hans Mark, director of the Ames Research Center, wrote that the analysis failed to take into account that programs sometimes were expanded in scope in midstream, as had happened in the Pioneer program. This, of course, also added to the total cost, but such increases were not properly classed as overruns. To get a true picture, one needed to take into account intentional changes in program.55
The Orbiting Astronomical Observatory was a good example of the kinds of trouble one could get into by trying to force too big a technological step. Some of the required subsystems for the satellite were not far enough along to ensure a smooth development of the spacecraft. The star trackers, for example, essential for establishing the stellar reference frame against which the spacecraft would be stabilized, ran into difficulties that took a long time to resolve. The cost of solving the problem was only part of the total increase, for, while engineers wrestled with the star trackers, a far greater number of workers on the rest of the observatory project also had to be paid as they waited for the star trackers.56
The observatory finally proved to be a powerful astronomical facility. But in retrospect it can be seen that NASA might have done better to follow the recommendations of its advisers, who would have preferred to start with a less ambitious astronomy satellite that would have permitted astronomical observations sooner. Having the less capable astronomy satellite sooner, the astronomers would have been content to wait for the larger one, as Edward Purcell and other members of the White House’s Space Science Panel had indicated.57
Both Ranger and Surveyor suffered from launch vehicle troubles. Five launch vehicle failures in a row impeded the development of Ranger, drawing the attention of NASA management and the Congress. When on the sixth flight the launch vehicle finally did work, sending the Ranger spacecraft precisely to the intended spot on the moon, only to have Ranger’s television system fail in the last seconds, the reaction was swift. Searching investigations into the engineering and management of the project were conducted both within NASA and by the Congress.58 The performance of the Jet Propulsion Laboratory and the Office of Space Science and Applications was under scrutiny, as well as that of the television contractor, Radio Corporation of America. The steps proposed to solve the problems were carefully examined by NASA management, and only the resounding success of Ranger 7 averted what might have been drastic management changes.
Surveyor’s launch vehicle troubles were of a more subtle kind. At the start the project suffered from lack of adequate management attention at both the Jet Propulsion Laboratory and the contractor, Hughes Aircraft Company. But Surveyor’s difficulties were exacerbated by simultaneous development of launch vehicle and spacecraft. The Centaur was the first major rocket to use the high-energy propellants liquid hydrogen and liquid oxygen. The Advanced Research Projects Agency had started Centaur in 1958 before NASA was created. When NASA took over responsibility, the project had been handled more as a research project than as a serious development effort, but NASA considered the Centaur stage an important Component of the launch vehicle stable.
Nevertheless, since only estimates could be given of the vehicle’s performance, it was difficult for Surveyor engineers to pin down weight and payload requirements for their spacecraft. These difficulties were further enhanced by the large number of other spacecraft being assigned to Centaur, influencing specifications for the rocket. In April 1962 Surveyor, Mariner, a variety of near-earth and synchronous-orbit missions, and the Department of Defense’s communications satellite, Advent, were assigned to the Centaur.59 The synchronous-orbit missions-that is, launchings to an altitude of 36000 km, where the satellite’s rate of revolution would equal the earth’s rate of rotation-would be about as demanding as missions to the moon.
When the program difficulties-including failure of the first flight test of Centaur-became too intense, NASA cut the Gordian knot by asserting that the initial Centaur would be developed for Surveyor only. Once Centaur had proved successful, a suitable program to uprate the vehicle could be instituted to meet additional requirements. Along with this decision, NASA also moved management of Centaur from the Marshall Space Flight Center-where the demands of Saturn preempted the center’s management attention-to the Lewis Research Center.60
But these changes did not come before Congress again had seen fit to delve into how NASA was performing. Congressman Joseph Karth’s Space Science and Applications Subcommittee of the House Committee on Science and Astronautics explored all aspects of Centaur: its management, its importance to the space program, its funding, the contractor, and even the engineering principles underlying the design.61 Such investigations into NASA failures made it perfectly plain that the agency had to bend every effort to avoid mishaps and provided much of the motivation for Associate Administrator Seaman’s policy, mentioned earlier, of seeking success on the first try. A variety of management tools was used to make the policy succeed. During the fall of 1963 and throughout 1964, much attention was given to devising and applying more effective management.62
For the development of the Polaris missile, the Navy had devised what was called the Program Evaluation Review Technique, or PERT, system.63 This laid out in graphical form the schedules of the different parts of a project to show how individual schedules were interrelated, and in particular to highlight the components or subsystems that were most likely to delay the whole project. Once highlighted, the critical elements of the project could be given the necessary funding and management and engineering attention needed to keep them moving in pace with the rest of the project. NASA adapted this system to its own projects.
Like the Department of Defense, NASA also experimented with special contracting devices to reduce cost overruns and delays in schedule.64 In the development of a new launch vehicle, spacecraft, or other equipment, it was not possible for either NASA or the contractor to specify in detail the product desired. Instead, performance specifications were given, and the technical approach then agreed on between NASA and the contractor. In this situation, the contractor was in no position to sign a fixed price contract. Instead, contracts were customarily “cost plus fixed fee,” that is, the government agreed to pay the actual allowable costs incurred in the development, plus a fee that was based on the contractor’s original estimate of what the costs would be. There was a certain amount of incentive in such an arrangement for a contractor to finish the work within the specified time and cost, for the longer a project ran and the higher the costs went, the smaller would be the percentage represented by the fee. Also, the performance of a company was bound to influence the government’s decision on the choice of contractors for other projects.
But experience showed that these incentives were not particularly effective in keeping costs down and schedules short. Therefore, additional incentives were introduced in the form of bonuses for meeting or beating schedules within estimated costs, and penalties for exceeding estimated costs and schedules.65 The value of such incentives was difficult to estimate precisely. Perhaps the greatest benefit lay in the increased management attention they evoked.
One of the most effective ways to keep costs down was to see that schedules were met. The longer a project ran, the longer salaries on the project were being paid, hence the greater were the total costs. Time was money, and if a project manager could keep to his schedule, he probably could keep close to his original cost estimates. A striking example was furnished by the 1967 Mariner mission to Venus, preparation for which began only a year or so before the launch date. Since the date for launching a spacecraft to a planet is fixed by the celestial mechanics of the solar system, there was no leeway in the schedule and the date had to be met. One result was that the project was completed for slightly less than the original cost estimate.66
Most projects had several distinct phases. First was the period in which the project was being conceived. The specific objectives would be worked out-for example, to investigate the solar wind and magnetic fields in interplanetary space. The feasibility of the project would be determined. Could appropriate experiments be designed and instrumented? Did a suitable spacecraft for the experiments exist or could one be made? Was there a suitable launch vehicle that could be used? Much of the effort during this phase would be paper work, supported by limited amounts of laboratory research.
A second phase would be that in which engineering studies analyzed various approaches, seeking, if possible, a best one. During this phase performance specifications were worked out, to provide the basis for detailed engineering design carried out in the third phase. Once the engineering design had been completed, it was possible to move into the fourth, final phase, that of actual development, in which hardware was made and tested. Major costs in the project would come during the fourth phase, and if delays occurred because engineering difficulties had not been properly anticipated during the earlier phases, large overruns could accumulate.
To try to avoid such overruns, NASA instituted what it called a “phased project planning system,” recognizing four phases A, B, C, and D, corresponding to those described above.67 The plan required completing the early phases before proceeding to later ones. The costs of phases A and B would be a minor part of the project expense, and one could afford to spend time at these stages getting matters right. Similarly, in the detail design phase, C, although costs would be appreciably higher than in phases A and B, they would still be far less than those of the construction phase, D. Again, it would pay to spend whatever time was needed in phase C to avoid problems later in phase D.
The phased project planning document was used in NASA less as a bible than as a set of useful guidelines. Wyatt’s study seemed to show that when the principles embodied in the phasing scheme were followed, costs and schedules were indeed kept in line.
By such devices NASA sought to sustain a high level of performance For its own use, the Office of Space Science and Applications held monthly status reviews, just before those of the associate administrator. At these reviews progress and problems were discussed for all parts of the program. Beginning in November 1966 these reviews, which had already been going on for several years, were documented in OSSA Management Reports.68
To keep a tight rein on the agency’s activities, the administrator required that the program offices obtain formal approval of all programs or projects. This process evolved over the years until in the latter 1960s a signed Project Approval Document-or PAD, as it came to be called-was required for each major element of the program.69 The PAD set forth the purpose of the project or program, outlined approaches to be taken, and gave estimates of schedules, manpower, and costs. The document described how the phases A, B, C, and D would be accomplished. Where outside contractors were to be used, a procurement plan acceptable to NASA’s legal and procurement offices had to be provided. Before a program office could obtain approval to move to the later phases of an approved project, the office in charge would have to furnish a detailed project development plan showing that the groundwork had been properly laid and that the path to completion of the project had been thought through. Administrator Webb liked to refer to the Project Approval Documents as contracts between him and his program managers. They furnished written evidence, for those who might wish to probe the agency’s performance, of the care that NASA took to ensure effective performance on its projects.
The wide scope of the space science program required a large number of space science PADs. Although these were primarily the concern of the program and project managers, they did affect the scientists themselves, in that experimenters were required to meet schedules, adhere to cost estimates, and furnish an appreciable portion of the documentation needed by managers to keep track of progress on their projects. Much of this was onerous to the scientists, who preferred to spend their time on their experiments. For manned spaceflight projects especially, where managers felt keenly the burden of ensuring absolute success, not only to justify the many dollars spent on the program but more importantly to protect the safety of astronauts, the schedules were quite rigid, and documentation considerably more detailed than for unmanned projects. Many scientists shied away from working in the manned program, preferring to fly their experiments in unmanned spacecraft for which the management requirements were less burdensome.
It is not likely that advanced research and development programs like those of NASA and the Department of Defense will ever be entirely free of mishaps and failures, cost overruns and schedule slips. Working at the frontiers of science and technology, the likelihood of encountering unforeseen technical difficulties must ever be present. The prescription for performing satisfactorily under such conditions is constant management attention and the most effective techniques for reducing the unforeseen to only the unforeseeable. In the space program, such management attention could produce acceptable performance. For launch vehicles, NASA’s performance improved over the years from a very poor showing in the first two years, to better than 90 percent successes in the 1970s (fig. 30).70 With spacecraft the success-on-the-first-try policy appeared to bear fruit. During the 1960s every Explorer satellite that was properly placed in space by its launch vehicle achieved its mission. The Orbiting Solar Observatory, Surveyor, Lunar Orbiter, Mariner, and Viking worked the first time out, as did the Canadian Alouette, the British Ariel, and the Italian San Marco. The first Orbiting Astronomical Observatory failed, but the second was highly productive. Like the astronomy observatory, Biosatellite was successful on its second flight. In spite of its hectic development history, the second time Ranger reached the moon it performed perfectly.
To complete the picture, applications satellites that succeeded right away included the Tiros and Nimbus weather satellites, the Echo, Syncom, and Intelsat communications satellites, NASA’s Applications Technology Satellite, and the geodetic satellite Geos. All the manned spaceflight spacecraft-Mercury, Gemini, Apollo, and the Lunar Module-worked on their initial manned flights.71 After more than a decade of experience, both NASA and the Department of Defense came to expect spacecraft to function correctly once they were launched properly, and with the increasing reliability of launch vehicles, the probability of success on a mission was very high.
With reliable launchers and spacecraft available to carry out their experiments, scientists could conduct a variety of experiments from near the earth to the remote regions of the solar system, and the value of what they did depended very much on their own scientific competence. Recognizing this, NASA had consciously set out to interest the best of the nation’s scientists in the space science program. Policies for supporting research, procedures for selecting experiments and experimenters, and arrangements for securing to NASA the best possible advice were designed to attract the best researchers into the program. Most important was the policy of letting the space science program become very much the creation of the scientists, with projects to attack the problems the scientists themselves perceived as the most fundamental and most likely to provide important new knowledge. The way in which this policy was put into practice is the subject of chapter 12. Before proceeding with the narrative, however, it is appropriate to review some of the significant space science results obtained in NASA’s first three or four years.
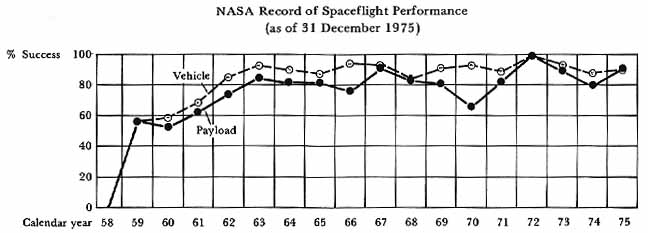
NASA success rate. After a poor beginning, NASA’s success rate rose steadily, eventually bettering 90 percent. NASA, “Historical Pocket Statistics" (Jan. 1972 and Jan. 1976); Astronautics and Aeronautics, 1972, NASA SP-4017 (1974), app. B.
Calendar year | 58 | 59 | 60 | 61 | 62 | 63 | 64 | 65 | 66 | 67 | 68 | 69 | 70 | 71 | 72 | 73 | 74 | 75 | Total |
NASA Payloads | |||||||||||||||||||
---|---|---|---|---|---|---|---|---|---|---|---|---|---|---|---|---|---|---|---|
Attempts | 4 | 14 | 17 | 24 | 27 | 13 | 30a | 28a | 29b | 22 | 18a | 16 | 9 | 11 | 10 | 10 | 5 | 13 | 300 |
Successes | 0 | 8 | 9 | 15 | 20 | 11 | 25 | 23 | 22 | 20 | 15 | 13 | 6 | 9 | 10 | 9 | 4 | 12 | 231 |
% successful | 0 | 57 | 53 | 63 | 74 | 85 | 83 | 82 | 76 | 91 | 83 | 81 | 66 | 82 | 100 | 90 | 80 | 92 | 77 |
NASA vehicles (some carrying non-NASA payloads) | |||||||||||||||||||
Attempts | 4 | 14 | 17 | 24 | 27 | 15 | 30 | 30 | 36 | 27 | 23 | 22 | 14 | 18 | 18 | 14 | 17 | 21 | 316 |
Successes | 0 | 8 | 10 | 16 | 23 | 14 | 27 | 26 | 34 | 25 | 19 | 20 | 13 | 16 | 18 | 13 | 15 | 19 | 371 |
% successful | 0 | 57 | 59 | 67 | 85 | 93 | 90 | 87 | 94 | 93 | 83 | 91 | 93 | 89 | 100 | 93 | 88 | 91 | 85 |
a Two missions launched on a single vehicle.
b Five missions on two vehicles are included.
c These figures include NASA vehicles used for non-NASA or cooperative missions.
Source Notes
- Homer E. Newell, Jr., Sounding Rockets (New York: McGraw-Hill Book Co., 1959); William R. Corliss, NASA Sounding Rockets, 1958-1968, NASA SP-4401 (Washington. 1971).X
- William H. Pickering, “History of the Juno Cluster System,” chap. 12 in Astronautical Engineering and Science, ed. Ernst Stuhlinger et al. (New York: McGraw-Hill Book Co., 1963).X
- Loyd S. Swenson, Jr., James M. Grimwood, and Charles C. Alexander. This New Ocean : A History of Project Mercury, NASA SP-4201 (Washington, 1966), pp. 21, 22, 341.X
- Constance McLaughlin Green and Milton Lomask, Vanguard: A History. NASA SP-4202 (Washington, 1970; Smithsonian Institution Press, 1971).X
- Ibid., p. 255.X
- Swenson et al., This New Ocean, chap. 13.X
- Green and Lomask, Vanguard, chap. 9.X
- As an illustration, see rpt. to Launch Vehicle Panel of Aeronautics and Astronautics Coordinating Board, “Intermediate-Class Launch Vehicles for Future DoD/NASA Manned Missions,” prepared by Ad Hoc joint DoD/NASA Vehicle Working Group, typescript, Nov. 1968.X
- Robert A. Goddard, “A Method of Reaching Extreme Altitudes,” in Smithsonian Miscellaneous Collections. vol. 71, no. 2 (Washington: Smithsonian Institution, Dec. 1919).X
- NASA, “A National Space Vehicle Program: A Report to the President" (Washington, 27 Jan. 1959); also Senate Committee on Aeronautical and Space Sciences. Investigation Of Governmental Organization for Space Activities, hearings before Subcommittee on Governmental Organization for Space Activities, 86th Cong., 1st sm., 24 Mar.-7 May 1959, pp. 12-24.X
- Wernher von Braun and Frederick I. Ordway III, The History of Rocketry and Space Travel (New York: Thomas Y. Crowell Co., 1966, rev. 1969). See also William R. Corliss, Scientific Satellites , NASA SP-133 (Washington, 1967), pp. 261-67.X
- Courtney G. Brooks, James M. Grimwood, Loyd S. Swenson, Jr., Chariots for Apollo : A History of Manned Lunar Spacecraft, NASA SP-4205 (Washington, 1979).X
- Roger E. Bilstein, Stages to Saturn : A Technological History of the Apollo/Saturn Launch Vehicles, NASA SP-4206, in press.X
- Robert L. Rosholt. An Administrative History of NASA, 1958-1967, NASA SP-4101 (Washington. 1966), pp. 72-76, 110, 213. In February 1962 NASA and the Department of Defense signed an agreement that neither agency would undertake development of a Space launch vehicle without first obtaining the written acknowledgment of the other. House Committee on Science and Astronautics, Astronautical and Aeronautical Events of 1962, 88th Cong., 1st sess., committee print, 12 June 1963, p. 21.X
- House Committee on Science and Astronautics, Review of the . Soviet Space Program, 90th Cong.. 1st sess., committee print, 1967, pp. 5- 10.X
- Arnold W. Frulkin, International Cooperation in Space (Englewood Cliffs, N.J.: Prentice-Hall, 134-37.X
- Ibid., p.45; Europe in Space (Paris: European Space Research Organization. 1974), . 180-81.X
- Air Force Systems Command, Air Force Eastern Test Range, a descriptive booklet prepared Office of Information, Hqs., Air Force Eastern Test Range, Patrick Air Force Base, Florida; Senate Committee on Aeronautical and Space Sciences, NASA Scientific and Technical Programs, hearings, 87th Cong., 1st sess., 28 Feb.-1 Mar. 1961, pp. 455-66; Henry R. Van Goey, “Western Test Range Operations Handbook" (Lompoc, Cal.: Kennedy Space Center, WTR, Jan. 1968); Helen T. Wells, Susan H. Whitely, and Carrie E. Karegeannes, Origins of NASA names, NASA SP-4402 (Washington, 1976), pp. 149-50.X
- Joseph A. Shortal, A New Dimension: Wallops Island Flight Test Range, the First Fifteen Years, NASA RP- 1028 (Washington, 1978).X
- Senate Committee on Aeronautical and Space Sciences, Soviet Space Programs, 1966-1970, staff report, 92nd Cong., 1st sess. (9 Dec. 1971), pp. 126-28.X
- R. R.Teeter, “Foreign Space Launch Vehicle Performance Document,” rpt. to NASA BMI-NLVP-DD-1(74) (Columbus. Ohio: Battelle Memorial Institute Columbus Laboratories. 8Apr. 1971). pp. 30-37.X
- Frutkin, International Cooperation, pp. 54-57, 145-46.X
- William R. Corliss, Scientific Satellites; idem, “NASA Spacecraft,” in the series America in Space/The First Decade (Washington: NASA, 1 Oct. 1968); idem, “Putting Satellites to Work,” ibid.; idem. “Mall in Space", ibid.; John E. Naugle, Unmanned Space Flight (New York: Holt, Rinehart & Winston. 1965); Max Faget, Manned Space Flight (New York: Holt, Rinehart & Winston, 1966), William K. Widger, Jr., Meteorological Satellites (New York: Holt, Rinehart & Winston, 1966): Brooks et al., Chariots for Apollo ; Wells et al., Origins of NASa names; James J. Haggerty, Jr., Spacecraft (Washington: National Science Teachers Association, 1961). NASA Facts: B-62, “Orbiting Solar Observatory”; C-62, “Ariel: First International Satellite”; E-10-62, “The Explorer Satellites”; F-12-62, “Alouette-Canada’s First Satellite”; G-12-62, “Project Relay”; A-1-63, “Explorer XVI: The Micrometeoroid Satellite”; Vol. 2, no. 1, “Interplanetary Explorer Satellites”; Vol. 2, no. 7, “Nimbus”; vol. 2, no. 8, “Manned Space Flight: Projects Mercury and Gemini”; Vol. 2, no. 9, “Mariner IV”; Vol. 2, no. 10, “Biosatellites”; Vol. 2, no. 12, “TIROS”; Vol. 2, no. 13, “Orbiting Geophysical Observatory”; Vol. 2, no. 14, “Project Syncom”; Vol. 2, no. 15, “Pegasus”; Vol. 3, no. 2, “Project Ranger”; Vol. 3, no. 4, “Explorer XXIX (The Geodetic Explorer)”; Vol. 3, no. 7, “Orbiting Solar Observatory”; Vol. 4, no. 1, “Gemini Pictorial”; NF-31, Vol. 4, no. 3, -The Pioneer Spacecraft”; NF-32, Vol. IV, no. 4, “Lunar Orbiter”; NF-35, Vol. 4, no. 6, “Surveyor”; NF-23, Vol. 4, no. 7, “Manned Space Flight: Apollo”; NF-39/2-68, “Mariner Spacecraft: Planetary Trailblazers". NF-43/1-72, “Skylab" (all Washington); Newell Files, box 40. Geophysics and Astronomy in Space Exploration, NASA SP-13; Lunar and Planetary Sciences in Space Exploration, NASA SP-14; Celestial Mechanics and Space Flight Analysis, NASA SP-15; Data Acquisition from Spacecraft, NASA SP-16; Control , Guidance, and Navigation of Spacecraft, NASA SP-17; Bioastronautics, NASA SP-18; Chemical Rocket Propulsion, NASA SP-19; Nuclear Rocket Propulsion, NASA SP-20; Power for Spacecraft, NASA SP-21; Electric Propulsion for Spacecraft, NASA SP-22; Aerodynamics of Space Vehicles, NASA SP-23; Gas Dynamics for Space Exploration, NASA SP-24; Plasma Physics and Magnelohydrodynamics in Space Exploration, NASA SP-25; Laboratory Techniques in Space Environment Research, NASA SP-26; Materials for Space Operations, NASA SP-27; Structures for Space Operations, NASA SP-28 (all Washington, Dec. 1962).X
- Samuel Glasstone, Sourcebook on the Space Sciences (New York: D. Van Nostrand Co., 1965). p. 31. Senate Committee on Aeronautical and Space Sciences, NASA Authorization for Fiscal Year 1961, hearings, 86th Cong., 2d sess., pt. 1. 28-30 Mar. 1960, p. 312.X
- Lloyd Berkner to George Kisdakowsky, 13 Nov. 1959. NF7(112); H. E. Newell. memo to file, conference rpt. of meeting of Space Science Panel of President’s Science Advisory Committee at Institute of Radio Engineers, New York, 18 Dec. 1959, NF12(173).X
- Charles D. Benson and W. D. Compton. A History of Skylab, in process; Edward C. Ezell and Linda N. Ezell. The Partnership: A History of the Apollo-Soyuz Test Project, NASA SP-4209 (Washington, 1978).X
- Joseph F. Saunders, ed., The Experiments of Biosatellite II, NASA SP-204 (Washington, 1971).X
- Newell, conference rpt. on meeting of Space Science Panel, 18 Dec. 1959, NF12(173).X
- Senate Committee on Aeronautical and Space Science, NASA Authorization for Fiscal Year 1976, hearings, 94th Cong., 1st sess., pt. 2, 18, 19 Mar. 1975. pp. 394-401.X
- Senate Committee, Soviet Space Programs , 1966-1970, pp. 198-209.X
- William R. Corliss, Histories of the Space Tracking and Data Acquisition Network (STADAN), the Manned Space Flight Network (MSFN), and the NASA Communications Network (NASCOM), NASA CR- 140.390 (Washington, June 197-1).X
- Corliss, A History of the Deep Space Network, NASA CR-151915 (Washington. I May 1976).X
- Corliss, Histories of STADAN, MSFN, and NASCOM.X
- "Areas of Research in the NASA Space Sciences Program,” 10 Feb. 1959, typescript; “The United States National Space Sciences Program,” 10 Feb. 1959. typescript. typing paper on plans for a biosciences program, 30 Mar. 1959; “NASA Program Planning on Space Sciences.” Apr. 1960. typescript; all NF2(33),-Senate Committee on Aeronautical and Space Sciences, NASA Authorization for Fiscal Year 1961, hearings, 86th Cong., 2d sess., pt. 2, Scientific and Technical Aspects of NASA Program, 30 June 1960, pp. 575-632.X
- Senate Committee, NASA Authorization for Fiscal Year 1961. pt. 2, Scientific and Technical Aspects of NASA Program, 1960, p. 786: idem, NASA Scientific and Technical Programs, 1961, pp. 31-35: Green and Lomask. Vanguard.X
- Eugene M. Emme, Aeronautics and Astronautics: An American Chronology of Science and Technology in the Exploration of Space, 1915-1960 (Washington: NASA, 1961), p. 102.X
- NASA, “Historical Pocket Statistics" (Washington, Jan. 1972). P. A-13.X
- Newell, memo for files, 16 Div. 1960, NF12(176).X
- J. A. Simpson to Newell, 30Aug. l96l, NF13(193).X
- Berkner to Kistiakowsky, 13 Nov. 1959, encl. p. 6, NF7(112), among other matters raised the question of backups for space science flights. NASA’s response to Berkner’s questions was given in T. Keith Glennan to Kistiakowsky, 3 Dec. 1959, NF7(112).X
- Glennan to Alan Waterman, 29 May 1959, NF11(171). Waterman, director of the National Science Foundation, no doubt prompted by the Space Science Board, had queried NASA as to agency policy on providing backups for its missions. See also Newell, conference rpt. on Space Science Board meeting, 11 Mar. 1960, typescript, NF6(106).X
- Green and Lomask, Vanguard, pp. 208-12.X
- Ibid., pp. 98-106. Author’s notebook, 30 Sept.-5 Oct. 1957, NF(28), records Richard Porter, U.S. delegate to CSAGI conference on rockets and satellites at National Academy of Sciences, Washington, as saying, in presenting the U.S. National Report, that America expects from Vanguard at least one success.”X
- Roger E. Bilstein, Stages to Saturn: A Technological History of the Apollo/Saturn Launch Vehicle, NASA SP-4206, in press.X
- Charles S. Sheldon II, United States and Soviet Progress in Space: Summary Data through 1972 and a Forward Look, 73-69 SP (Washington: Library of Congress, 29 Jan. 1973). pp. 2-3.X
- Ibid., p. 3.X
- Senate Committee on Appropriations, Independent Offices Appropriations, 1961, hearings before Subcommittee, on H.R. 11776, 86th Cong., 2d sess., 1960, p. 276.X
- House Committee on Science and Astronautics, 1966 NASA Authorization, hearings before the Subcommittee on Science and Applications, 89th Cong., 1st sess., pt. 3,4-26 Mar. 1965, pp. 803-06.X
- Brooks et al, Chariots for Apollo, Senate Committee, Soviet Space Programs, 1966-1970, pp. 230-32.X
- House Committee on Science and Astronautics, Investigation into Apollo 204 Accident, hearings, 90th Cong., 1st sess., vols. 1-3,10 Apr.- 10 May 1967.X
- NASA Historical Div., Astronautics and Aeronautics, 1968: A Chronology on Science, Technology, and Policy, NASA SP-4010 (Washington, 1969), p. 157.X
- Idem, Astronautics and Aeronautics, 1964, NASA SP-4005 (1965), p. 135.X
- Merton J. Peck and Frederic M. Scherer, The Weapons Acquisition Process. An Economic Analysis, (Boston: Harvard, Grad. Sch. of Bus. Admin., Div. of Res., 1962), p. 22.X
- D. D. Wyatt to NASA administrator, “NASA Cost Projections,” 10 Apr. 1969, NF40.X
- Hans Mark to Wernher von Braun, 22 Apr. 1971, NF40.X
- House Committee, 1966 NASA Authorization, pp. 529, 1090-91.X
- Newell, conference rpt., Space Science Panel, 18 Dec. 1959, NF12(173).X
- R. Cargill Hall, Lunar Impact: A History of Project Ranger, NASA SP-4210 (Washington, 1977). pp. 252-54.X
- NASA chart on Centaur mission assignments. 27 Apr. 1962, NF72(32).X
- NASA, Office of Space Science and Applications, “Atlas Centaur Development Flight,” Mission Operation Rpt. S-891-63-01 (Washington, 22 Nov. 1963), pp. 8-9.X
- House Committee on Science and Astronautics, Centaur Program, hearings before Subcommittee on Space Science and Applications. 87th Cong., 2d sess., 15, 18 May 1962, and Centaur Launch Vehicle Development Program, H. rpt. 1959, 2 July 1962.X
- Arnold Levine, Managing NASA in the Apollo Era, in process.X
- Ibid.X
- Ibid.X
- Ibid.X
- Wyatt to NASA admin., “NASA Cost Projections.”X
- Arnold Levine, Managing NASA.X
- NASA, OSSA Monthly Status Reports (Washington: Office of Space Science and Applications, NASA, Nov. 1966.); NASA Issuance NHB 2340.2, -OSSA/OART Project Management Information and Control System (MICS)" (Washington, 1 Nov. 1966), formalized publication of reviews of the status of projects which had been conducted monthly for the past three years.X
- Arnold Levine, Managing NASA.X
- NASA. “NASA Record of Performance" in “NASA Pocket Statistics.” (Washington, Apr. 1976). p. 5.X
- NASA, “Historical Pocket Statistics" (Washington, Jan. 1972). pp. A-9 to A-11; ibid. (Jan. 1976). pp. C-3, C-5.X